Removal of PFAS by Biological Methods
Download
Abstract
The ubiquitous presence of poly- and perfluoroalkyl (PFAS) is a severe concern in view of their bioaccumulation and persistence in the environment. Subsequently, through feeding or drinking contaminated water, this contaminant will enter the body of living organisms and humans and will cause serious diseases, specifically, different types of cancers. There are many chemical, physical and biological methods used for PFAS removal. However, besides some limitations, biological methods are one of the most cost-effective, eco-friendly, and simplest in operation process. Biological techniques include bioremediation, phytoremediation, vermiremediation, biodegradation, and bioadsorption, comprehensively reviewed in this study. Since combination of different techniques are more effective and efficient than a single method, we also reviewed different kinds of combination methods.
Introduction
Perfluorochemicals (PFCs) is often used interchangeably with perfluoroalkyl (PFASs), although in fact, it refers to a larger group that includes perfluoropolyether. These are persistent organic pollutants that are commonly found in the environment due to their resistance to degradation [1]. Per- and polyfluoroalkyl substances (PFAS), also known as forever chemicals are ubiquitous environmental pollutants and are regarded as a new anthropogenic type of carcinogenic contaminant that remains untreated by conventional treatment methods. These contaminants are typically resistance to biological and chemical degradation, non-hydrolysable, and poses many risks to environmental safety and human health. PFASs are mainly fluorinated anionic surfactants, consisting of straight-chain or branched alkyl groups, with more than 3,000 synthetic organic chemicals made and used since the 1950s [2-4]. The well-known PFAS types are perfluorooctane sulfonate (PFOS) and perfluorooctanoic acid (PFOA), that the unique combination of hydrophobicity and hydrophilicity in their structures allows them to have superb surface performance and enable them to be widely used in various industrial applications, thus they are still being produced in large quantities, and are overlooked [2-4]. PFOA and PFOS absorbed into the body can bioaccumulate and lead to chronic diseases such as testicular and kidney cancers and ulcerative colitis [5]. Moreover, PFASs are perdurable pollutants that may cause breast cancer. However, the link between PFAS exposure and breast cancer risk is a controversial debate. However, PFASs may be potential risk factors for breast cancer, and compounds at low exposure levels can have more harmful effects on human health [6-10]. Most evidence has shown the association of PFAS with testicular and kidney cancers. A few studies have also suggested an association with prostate cancer, but the data are conflicting [11, 12]. These compounds have unique chemical and physical properties that allow them to be used in firefighting efforts, non-stick surfaces, as soft coatings, and other applications. However, recent concerns about the health effects of these compounds have led to increased international community research and attention to their degradation methods [13].
Perfluoroalkyl materials, structurally, are composed of anionic, cationic, and zwitterionic species that interact differently with soil and water. Due to their high-water solubility, PFASs are highly mobile in soil and water environments and can travel long distances from places of use or production even to the polar regions [14]. Therefore, it leads to off-site contamination and it is difficult to remove PFASs from the environment. In fact, it is difficult to fully decompose PFAS into their basic elements, and this is one of the major limitations of currently available PFAS remediation methods [15]. The general structure of PFASs with carbon backbones of different lengths with hydrogen atoms that have been completely replaced by fluorine atoms [16] is shown in Figure 1.
Figure 1. General Structure of PFASs where X Represents a Hydrophilic Functional Group Such as Sulfonates (–SO −) and Carboxylates (–COO−) (Benford et al., 2008).
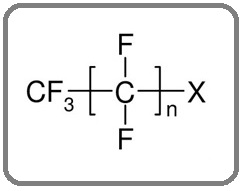
Introduction to PFAS remediation technologies
Removal of PFASs is a substantial issue that, if not given enough attention, the next generation will face serious problems. Remediation generally focuses on surface water, drinking water, soil, sludge, and sediments where contaminants are widespread. Typical modification of PFAS includes adsorption, advanced oxidation, physicochemical processes, advanced reduction processes, biological treatment, and other treatment processes such as ozone and electron beam [18]. This review has the novelty of investigating the various biological methods and hybrid approaches to PFAS removal. It also aims to provide future perspectives for PFAS biological treatments.
PFAS biological treatment
Although biological approaches have the potential to eliminate PFASs, very few scientific papers have been focused on the study of bioremediation for this purpose. Different chemical and biological methods can be used to remove these contaminants, but the use of biological treatment methods is a better and less dangerous approach to remove them than chemical methods. Bioremediation of PFAS using naturally occurring microbial degradation pathways represents a novel approach to remove PFAS contamination [13]. On the other hand, biological treatment or bioremediation has been prioritized due to the low possibility of producing secondary pollutants and cost-effectiveness in removing PFASs [19, 20].
There are two main category of bioremediation including microbial bioremediation and Phytoremediation. Former refers to using microorganisms or their derivatives to clean-up environmental contaminants, but the latter is one of the remediation methods, using plants with certain advantages, such as being cost-effective, flexible, less harmful, and efficient in removing PFAS [21]. In addition, vermiremediation as a form of bioremediation is also the process of biodegradation of organic matter through the interactions between earthworms and microorganisms [22]. Furthermore, in biodegradation processes, a chemical compound is transformed or eliminated by the biological action of living organisms. In general terms, biodegradability is the inclination of a substance to be ingested and metabolized by microorganisms [23]. Other approach for the removal of PFAS is bioadsorption which is an eco-friendly and economic approach. This technique is broadly used to remove different classes of pollutants [24].
This review study has two main objectives. The first is to provide an overview of biological methods including bioremediation, phytoremediation, biodegradation, and bioadsorption methods to reduce or eliminate PFAS in multiple environmental matrices. The second is a comprehensive review of using a combination of various methods for the removal and to provide future perspectives. Hence, the overall aim is to provide a wide perspective of the feasibility and efficiency of biological methods in PFAS removal from the environment.
PFAS Bioremediation
Remediation of soils contaminated with organic pollutants has become an urgent requirement worldwide. Bioremediation, the use of living organisms to remediate contaminated sites, is an alternative approach that is considered a cost-effective and environmentally friendly method, but its main limitation is the bioavailability of the organic pollutants in the soil. However, several organic solvents such as cyclodextrins have been proposed to increase soil organic bioavailability and enhance soil bioremediation [25]. Although bioremediation and biodegradation are promising methods for the removal of organic pollutants, biodegradation processes for PFAS treatment are underdeveloped currently. Extremely long reaction times, a very slow decomposition and degradation rates, low removal rates, the long half-life of precursors, and great different mass balance based on environmental conditions hinder the feasibility and scalability of such processes [26]. The limited research on biodegradation of PFAS has shown partial transformation of PFAS with several unknown products. More in-depth bioremediation studies on PFAS, especially, focusing on defluorination are required. So far, no organism has been found that can completely defluorinate PFAS, but researchers have no reason to believe that microbes couldn’t eventually do the job. In the laboratory, researchers can create ideal conditions for microbes to feed on PFAS or even force them to do it. Deploying microbes out in the environment for in-situ remediations, however, presents significant challenges [27].
The recently discovered microbial reductive defluorination of unsaturated fluorinated carboxylic acids (FCAs) provided valuable insights into the environmental fate of PFASs and potential bioremediation strategies. However, a systematic review is needed to further demonstrate the role of C═C double bonds in the biodegradability of unsaturated PFASs. Indeed, Such FCA structures can undergo anaerobic abiotic defluorination in the presence of reducing agents and significant aerobic microbial defluorination. Given the various applications and emerging concerns of fluorochemicals, this work not only advances the pivotal understanding of the fate of unsaturated PFASs in natural and engineered environments but also may provide insights into the design of readily degradable fluorinated alternatives to existing PFAS compounds [28].
Regarding the bioremediation of PFAS, Tang and Kristanti (2022) [29] represents that multiple Pseudomonas sp. could degrade perfluorochemicals particularly perfluoroalkyl acids under aerobic condition. Acidimicrobium sp. degraded perfluoroalkyl acids anaerobically in the presence of electron donors. It was concluded that a mixed of Pseudomonas culture was more effective than pure cultures. The study of Senevirathna et al (2022) [30] also investigates the behavior of bacterial communities that inhabit PFAS-rich soil. 11 genera showed significant correlations (P<0.05) with soil PFAS levels. They indicated that the diversity and population of soil bacteria are adversely influenced by PFAS. The dominant PFAS detected in soil samples was PFOS, which accounted for 82% of total PFAS and the maximum PFOS level was noted in the topsoil. Regardless of the degree of PFAS contamination at different depths, a comparable amount of each PFAS was observed in soil samples. Based on their study, a remarkably higher diversity of bacterial sequences were identified in uncontaminated soil than PFAS contaminated soil. Bacterial genera and Chujaibacter were dominant in the PFAS contaminated soil. Three different bacterial genera including the genus Chujaibacter of Gammaproteobacteria, Ambiguous taxa of Acidobacteriia, and Alphaproteobacteria showed a significant positive correlation. Overall, the results suggest that the counts and species diversity of soil microorganisms are adversely influenced by PFAS contamination [30].
In case of using synthetic biology as a bioremediation approach, Marco et al. (2021) [31] proposed Phyco- remediation as a green alternative approach to wastewater treatment. In this study, the possibility of using Synechocystis sp. PCC6803, a unicellular photosynthetic organism, was evaluated as a candidate for bioremediation of PFAS-enriched water. Based on the results, partial elimination was observed for PFOS and PFOA (88% and 37%, respectively). It has also been shown that PFOS is predominantly internalized in the cell, while PFOA is somehow transformed by still unknown pathways. A preliminary bioinformatics search could point to transporters and enzymes that might be involved in such transformation processes. Moreover, according to the study of Jaffe et al (2018), a novel anaerobic bioremediation system is capable of degrading wide range of organic pollutants, including halogenated organics, PFAS, PFOA, and aromatics to harmless intermediates.
Microbial PFAS degradation
The microbial cleavage of fluorinated alkyl compounds requires at least one hydrogen atom in the alkyl chain for primary attack to occur. The additional difficulty to oxidatively replace fluorine atoms lies in their ability to form a dense hydrophobic layer surrounding carbon-carbon bonds, preventing oxidative degradation. This characteristic fluorine-saturated carbon chain element provides resistance from oxidation under environmental conditions or utilization by microorganisms as carbon and energy sources. Initial anaerobic and aerobic biodegradation studies showed decreasing PFOA and PFOS concentrations, but were likely attributed to ongoing sorption processes. A limited number of studies have tested microbial degradation of PFASs and many conflicting reports exist, all suggesting that more research needs to be conducted to fully understand the biotic transformations of those compounds.
Several bacterial strains have shown to degrade PFASs under aerobic conditions. For instance, Pseudomonas strain D2 partially degraded sulfonates with hydrogen, such as H-PFOS and 2,2,2-trifluoroethane by defluorination under aerobic, sulfur-limiting conditions. In another study, Pseudomonas butanova was able to degrade 6-2 fluorotelomer alcohols (6-2 FTOH) precursor. Moreover, Actinobacteria may be able to grow on 8-2 FTOH.
A handful number of studies testing microbial degradation of PFASs have yielded conflicting reports, suggesting that more work needs to be performed to fully understand the biotic transformations of PFASs. Anaerobic transformation of which has also been investigated although the focus has been on polyfluorinated substances. For instance, an increased leaching of PFASs and detection of known biodegradation intermediates in anaerobic bioreactors filled with municipal solid waste relative to abiotic controls have been recorded. Another opposite study concluded that pure culture Desulfovibrio desulfuricans did not degrade PFOS in short-term or long-term experiments.
Regarding the PFAS biodegradation, according to the study of Ruiz-Urigüen et al (2022) [32], PFOA was degraded in microbial electrolysis cells (MECs) seeded with Acidimicrobiaceae sp. strain A6. Ammonium was the electron donor for the growth of A6 and reduction of PFOA. An average of 77% decrease in PFOA concentration in the active MECs was observed. In MECs were PFOA decreased, fluoride as well as smaller PFAAs where produced. In the study of Huang et al (2022) [33], PFOA in biosolids was degraded anaerobically by Acidimicrobium sp. Strain A6 (A6). Total, dissolved, and sorbed PFOA concentrations decreased with incubation time. Degradation of PFOA in biosolids required augmentation with A6 and ferric iron. Increasing PFOA concentrations affected the microbial community composition.
Furthermore, based on the study of Sáez et al (2008), bacterial communities from sewage sludge were exposed to a mixture of PFAS under aerobic or anaerobic conditions. No conclusive evidence for PFAS degradation was observed under the experimental conditions tested in this work. Reduction in concentrations, however, was observed for some PFAS in sludge under aerobic conditions. Moreover, in the study of Huang and Jaffé (2019), removal of up to 60% of PFOA and PFOS was observed during 100-day incubations, while total fluorine remained constant throughout the incubations. Results showed that Acidimicrobium sp [34]. Strain A6 can defluorinate PFOA/PFOS while reducing iron, using ammonium or hydrogen as the electron donor.
Fungal PFAS degradation
Some research on the fungal degradation of PFAS has been carried on owing to the wide range of substrate reduction catalyzed by extracellular ligninolytic enzymes. In this regard, experiments with white-rot fungus Phanaerochete chrysosporium exposed to FTOH under aerobic conditions expressed a significant decrease (45%) in concentrations over a duration of 35 days with the production of several shorter-chain metabolites such as perfluorobutyric acid (PFBA) and perfluoropentanoic acid (PFPeA). Another project explored PFOA degradation during enzyme-catalyzed oxidative humification reactions (ECOHR) that are catalyzed by lignin peroxidase, manganese peroxidase, and laccase. ECOHR-mediated PFAS degradation resulted in a 30% reduction in PFOA concentrations. Another application of fungal enzyme treatment is the use of enzymecatalyzed oxidative coupling (ECOC) reactions. Elementary results illustrated decreases in PFOS and PFOA levels in soil via laccasemediated ECOC with a lesser reduction percentage than in aqueous systems, suggesting that PFAS adsorption to soil particles may be responsible to some extent for these removal rates. Laccase catalyzed PFOA degradation during ECOHR (over 30% PFOA mineralization) and GAC immobilized laccase show promise for potential remediation applications (25% reduction). The innovative delivery of fungal enzymes for PFAS treatment entails further research.
Regarding the fungal degradation of PFAS, based on the study of Merino (2016), Phanerochaete chrysosporium, a wood-decaying fungus, transformed 6:2 fluorotelomer alcohol (6:2 FTOH), towards less fluorinated polyfluorocarboxylic acids. Gloeophyllum trabeum, Trametes versicolor, and several fungal isolates were able to transform 6:2 FTOH towards 5:3 acid. While most fungal pure cultures were limited in their ability to transform 6:2 FTOH under the nutrient conditions tested, the resulting metabolites will likely be further transformed in the environment via other biotic or abiotic processes. These studies demonstrate that fungi possess unique biochemical pathways for degrading polyfluoroalkyl substances towards more biodegradable and less fluorinated products [35].
Merino et al (2018) in another study, investigated the fungal degradation of six fungal isolates from a site contaminated with PFASs [36]. This study indicated that fungal pathways should be considered for the biodegradation of potential PFAS precursors, such as 6:2 FTOH, and proposed a basis for selecting appropriate microorganisms to remediate fluoroalkyl-contaminated sites. Besides, in the review by Tang and Kristanti (2022), fungal species, particularly Pseudeurotium sp. and Geomyces sp. revealed the potential to degrade perfluorooctanoic acid or perfluorooctane sulphonic acid [29].
Algal and microalgal PFAS biodegradation
Biodegradation or biotransformation, of ECs (Emerging Contaminants) by microalgae provides one of the most promising technologies for the remediation of contaminants of concern. Unlike bioadsorption or bio-uptake, which simply acts as biological filters to concentrate the EC and remove it from the surrounding aqueous solution, biodegradation involves the transformation of complex compounds into simpler breakdown molecules through catalytic metabolic degradation. Biodegradation overcomes any issues associated with the disposal of EC-laden microalgal biomass that is generated during bioabsorption and bio-uptake treatment. Microalgal biodegradation can occur via two principle mechanisms; either by metabolic degradation, in which the EC serves as the carbon source or electron donor / acceptor, for the microalga, or by co-metabolism, in which the EC is degraded by enzymes that are catalyzing other substrates present.
Microalgal biodegradation of ECs such as PFAS involves a complex enzymatic process that involves both Phases I and Phase II enzyme families. Phase I of the biodegradation involves cytochrome P450 enzymes, which include monooxygenase, dioxygenase, hydroxylase, carboxylase, and decarboxylase enzymes. In biodegradation, the main role of these enzymes is to make a contaminant more hydrophilic, through the addition, or unmasking of a hydroxyl group through either hydrolysis, oxidation, or reduction reactions. In Phase II, enzymes, such as glutathione-S-transferases, catalyze the conjugation of glutathione with a wide group of compounds possessing electrophilic centres, resulting in the opening of the epoxide ring to protect against oxidative damage in the cell.
Regarding the algal degradation of emerging contaminants, Algal Turf ScrubberTM (ATS) systems have been widely used for biomass production and pollutant removal through periphytic algae, but have not been yet studied for PFAS. The project of Lopes Viticoski (2019) [37] evaluated the suitability of an ATS approach for the remediation of a mixture of PFAS (PFOS, PFOA, PDHA, and HFPO-DA), and quantified the mechanisms of remediation. The amount of contaminants found in the biomass was equal to 1.24 ± 0.40% for PFOA, and 1.21 ± 0.41% for PFOS based on their initial concentration. In contrast, it was observed that between 35-92% of the initial concentration of PFOS and PFOA remained unaccounted after the mass balance was performed. Finally, it was concluded that ATS might not be an effective alternative for PFAS remediation, due to the low removal rates. Nevertheless, results from this analysis can contribute to the growing understanding of the bioaccumulation potential of these compounds.
PFAS Phytoremediation
PFAS are relatively soluble and remain in the soil solution, leading to their easy uptake by plants and subsequent removal using phytoremediation technology. The plant uptake of PFAS compounds is dependent on their chain length and the sorption behavior of soils. Promising PFAS compounds suitable for phytoremediation include those with a small C chain [38]. In recent years, our understanding of PFASs as contaminants in vegetation has extremely increased. PFASs are taken up, transformed, and accumulated in various portions of plants, and the rate of these processes depends on environmental conditions, compound-specific characteristics, plant species, and other competing factors [39].
PFASs that enter the plant are distributed by transpiration in different parts of the upper and lower parts of the plant, resulting in their bioconcentration in various parts of the plant. Studies have shown that the bioconcentration of long-chain PFASs is relatively lower due to their higher hydrophobicity, which prevents them from being absorbed by the roots [40, 41]. In addition, PFAS with longer carbon chains tends to bioaccumulate in the roots through adsorption on the root surface due to their higher hydrophobicity [42].
Contrast
For removal of organic pollutants, corresponding mechanisms of phytoremediation generally consist of phytofiltration (plant uptake), phytovolatilization (conversion of pollutants to volatile form), and phytodegradation (participation of root exudates and microbial populations) [43-45]. Phytoremediation of a PFAS contaminated site can be a slow process, but it involves low capital cost and almost no maintenance cost. Thus, phytoremediation states as the most economic and sustainable green technology available for the remediation of PFAS contaminated sites [46].
Plant uptake mechanisms suggested that PFASs from the soil or aquatic environment were frequently carried into plant roots through both passive and active processes. The passive transport is mostly dependent on the diffusion of small-molecular and non-ionized PFASs and the driving force of the transpiration stream. When PFASs pass through the root apoplast via diffusion, they will eventually be translocated to the aboveground parts (i.e., stems, leaves, shoots, and fruits) of plants through the transpiration stream. On the contrary, the active transport of PFAS is commonly attributed to selective adsorption by specific transporters such as ion channels and aquaporins to transport ionized PFASs into plant cells. Moreover, airborne PFASs and homologs presented in the phase of vapor and particulate could be adsorbed into plants via aerial parts such as foliage and barks [47]. Trees and wetland plants may be used as phytoremediation plants to remove PFASs from soil and water, because of their extensive root systems and large biomass, although the effectiveness likely varies among plant species as well as types of PFASs [6].
Regarding the removal of PFAS using plants, Gobelius et al (2017) reported successful uptake of 26 PFAS compounds in plants from contaminated soils around a firefighting training site in Stockholm [43]. Besides, in the study of Huff et al (2020), a greenhouse study evaluated the potential of seven woody and eight herbaceous plant species to absorb PFAS compounds. considerable accumulation of all PFAS compounds occurred in at least one plant species [48]. Mass recovery in aboveground tissue by the best plant varied from 3.8% for PFOS by Festuca rubra (Red Fescue) to 42% for PFPeA by Schedonorus arundinaceus (Tall Fescue). The results show the potential use of phytoremediation as a tool to modify PFAS- contaminated sites. They also evaluated the bioremediation of PFCs with different types of plants including Cynodon dactylon (Scutch Grass), Equisetum hyemale (Rough Horsetail), and Helianthus annus (Common Sunflower).
Moreover, four different treatments with different amendments (fertilizer and microbial fertilizer) were applied to the plants in the study of Wu (2021) [21]. The results indicated that PFASs were mostly transported and accumulated in the leaves, as opposed to the other plant compartments. Hemp had the highest levels of PFASs in the plant tissue (14.3 μg/plant) in comparison to sunflower (12.9 μg/plant) and mustard (8.3 μg/plant) in all control samples. Even though the total uptake of PFASs by mustard is the lowest, the PFAS concentration in mustard leaf is the highest (1.2 μg/g dry weight (dw)) among all plant compartments. The amendment with nutrient fertilizer and the amendment with microbe fertilizer decreased the PFAS concentration in the plant tissue, due to the sorption between PFASs in the soil and the fertilizer added. In conclusion, hemp seems a promising candidate for phytoremediation of PFAS contaminated soil.
In addition, based on the study of Zhang et al (2019b) [49], uptake of PFAAs by Juncus effusus increased with increasing PFAAs exposure concentration and time. PFOS was largely accumulated in the roots with limited upward translocation and PFAAs with shorter carbon chain length tended to accumulate in plant shoots. Also, according to the study of Tang and Kristanti (2022) [29], several plants were found to bioconcentrate perfluorochemicals and many revealed the ability to hyperaccumulate perfluoroalkyl acids, especially Festuca rubra, Salix nigra, and Betula nigra. In another study, Yin et al (2017) [50] studied the removal of PFAS in a full-scale tropical wetland, in which PFASs were removed up to 96% largely by plant uptake. They discovered the capacity of straw substrates to absorb PFAS. However, the effectiveness of this method must be confirmed in the field scale for remediation of PFAS-contaminated soils. This method also requires the removal of PFAS from plants.
In the study of Zhang et al (2019c) [40], the transport pathways of perfluoroalkyl acids (PFAAs) by wheat (Triticum acstivnm L.) have investigated. They also studied the uptake of five perfluoroalkyl carboxylic acids (PFCAs) using hydroponic experiments. Results elucidated that the uptake and translocation of PFAAs in wheat depended on their carbon chain length. Accumulation of PFCAs in wheat shoots decreased with their carbon chain length. The energy-dependent active process was the main mechanism for the uptake of PFAAs. Aquaporins and anion channels also contributed to the uptake of C2 and C3 PFCAs. Wang et al. (2019) [51] found that among submerged plants, P. crispus had the highest mean levels of PFAA, followed by V. natans, C. demersum, and Ulothrix. The study by Wang et al. (2016) [52] also reported that for emerged plants, N. nucifera and J. serotinus had higher levels of PFAA than other plants. C. demersum and V. natans also had the highest bioaccumulation factors (BAFs) for PFOA and PFOS, respectively.
In case of crop study on bioaccumulation of PFAS, Krippner et al (2015) [53] demonstrates that food crops such as wheat and maize can accumulate PFAAs from spiked soils with different parts of the crop that exhibit different BCFs. Maize straws showed the ability to over-accumulate PFBA and PFAA concentrations in maize straws were generally significantly higher than maize kernels. In the case of wheat, the BCFs of PFAAs for grains and husks were low even at the highest levels of contamination [54]. These studies represent that bioconcentrations vary in different parts of the plant and are affected by PFAS levels in the soil, which is true for most contaminants. The use of crops, especially food crops, for the biological purification of PFAS carries the risk of transferring contaminants to food chains. Therefore, they are not usually used in studies that deal with bioremediation [55].
phytoremediation is likely the most sustainable and desirable option because it is relatively inexpensive and also less destructive to the PFAS contaminated site in spite of its requirement for a long-time interval. Further work is needed to identify more efficient plant species for PFAS phytoremediation and removal [39].
PFAS vermiremediation
The use of earthworms to remove soil organic pollutants (e.g., PFAS) is a common bioremediation method. However, evaluating and predicting their impact on the removal of soil organic pollutants based on earthworm toxicology and pollutant degradation rates is a challenging issue. Meta-analysis studies also showed that the average effect of earthworms on the degradation of organic pollutants is 128.5%. In addition, soils with high organic matter or clay texture are more conducive to the removal of organic contaminants using earthworms [56]. In the study by Karnjanapiboonwong et al. (2018) [57], earthworms were exposed to PFAS-contaminated soil for 21 days. No earthworm mortality was observed in all treatments including control, except for all PFAS at 100,000 μgkg-1. Furthermore, the results are expected to fill some data gaps in the toxicity of PFASs in terrestrial environments and provide advantageous information on the nutrient transfer potential of PFASs from soil to higher organisms [57]. For this reason, there are some different adsorbents in studies that can prevent the accumulation of PFASs in earthworm bodies. For instance, in study of Jarjour et al (2022), stabilization using modified clay adsorbents to reduce PFAS bioaccumulation by earthworms (Eisenia fetida) was investigated in comparison with coal-based activated carbon. Both adsorbents reduced the PFAS load in the earthworm body at the end of the 28-day uptake phase. The highest concentration of adsorbent (4 w / w%) was the most effective and reduced PFAS body burden by more than 95%. The final results revealed that the modified bentonite adsorbents reduced PFAS leaching and bioaccumulation by earthworms. In another study by Melo et al. (2022) [58], a new adsorptive organoclay (Intraplex A®) was developed to stabilize PFAS in situ in the vadose zone. However, soils with Intraplex A® had negative effects on plant growth and earthworms, which should be balanced with its benefits as an in-situ PFAS adsorbent. Continuation of these studies assists to gain a deeper understanding of the potential of using earthworms to reduce soil organic contamination and to develop earthworm-based soil remediation techniques all around the world [56].
Biomaterials and bioadsorption
Bioadsorption is a complex process that involves different interaction mechanisms such as adsorption, ion exchange, complexation, and precipitation. Biological materials suitable for biosorption processes can include microbial biomass, agricultural waste, industrial by-products, or natural materials, which due to their high efficiency, low cost, and high abundance, have been introduced as promising substrates for the removal of pollutants by biosorption [59]. In the sorption and stabilization technique (usually called immobilization), sorbents are added to the soil and mixed with it to stabilize and immobilize the PFAS compounds within the soil. This reduces the potential of PFAS leaching to the groundwater. The sorbent adsorbs PFAS so the concentration in the liquid phase is decreased. This remediation option has been commercialized and applied in many contaminated sites across the world [60].
The adsorption of contaminated materials by adsorbents is one of the non-degradative treatment technologies for PFAS contaminated environments that is also cost-effective, less toxic, eco-friendly with the simplicity of design, high removal efficiency, and easy recyclability [61, 62]. Different sorbents have been used in the laboratory as well as in the field. These include activated carbon (AC) (powdered, PAC or granular, GAC), resins, minerals, biomaterials, molecularly imprinted polymers, etc [60]. Application of natural adsorbents such as biomaterials and minerals are attractive alternatives due to their abundance, low commercial value, and environmental sustainability, but they also have restrictions due to their use of energy for off-site disposal and incineration after single use or reconstruction [63].
For instance, Biochar (BC) material, as a novel and environmentally friendly adsorbents, is the carbon-rich product obtained when agricultural wastes, wood, manure or leaves, are produced under inert condition, and at elevated temperatures [64]. They have recently been explored as alternatives to Granular Activated Carbon (GAC) for PFAS removal from the environment [65]. Biochar can extensively be utilized for the remediation of contaminated soils and water in the last decade [66]. Biochar adsorbent can be produced in low-resource settings using local materials and simple pyrolysis technology, and it has shown promise for uptake of micropollutants such as pesticides, pharmaceuticals, industrial compounds, and chemicals released from consumer goods present in soil and water. Accordingly, the use of BC in water treatment applications where GAC is economically or logistically impossible has been considered [67].
The specific nano-treatment can improve the distribution and properties of biochar [68]. Nano-biochar is nanosized biochar with better physical, chemical and surface properties. It has numerous advantages such as improvement in plant growth and soil properties, bioremediation of contaminants and pesticides, treatment of wastewater and so on. It has excellent capability of adsorbing pollutants and nutrients [69]. Several nanomaterials, comprising inorganic oxides such as silicon, iron, aluminum, and titanium oxide, and carbon-based nanomaterials, namely multiwalled carbon nanotubes, and graphene-based nanomaterials have been studied as nano-sorbents for the removal of PFAS via a non-degradative approach [63].
Regarding the bioadsorption of PFAS and particularly by using biomaterials sush as (nano)biochar, Vo et al (2022) [70] aimed to understand the influences of PFASs properties and groundwater chemistry to PFASs sorption by BC and they demonstrate that sorption of PFSAs to BC was 1.3- fold higher than that of perfluoroalkyl carboxylates (PFCAs). Bsides, According to Kundu et al (2021) [71], It was concluded that >90% removal of PFOS and PFOA from biosolids derived biochar could be achieved in the pyrolysis–combustion integrated process. The biosolids derived BC showed >80% adsorption of long-chain PFAS and 19–27% adsorption of short-chain PFASs from water.
Steigerwald et al (2021) [65] studied on adsorption behavior of PFOS onto activated spent coffee grounds BC in synthetic wastewater effluent. The results indicate that BC surface area was greatly increased by potassium hydroxide activation and the presence of simulated effluent organic matter decreased PFOS removal. In this regard, Liu et al (2021) [72] also synthesized reed straw-derived biochar (RESCA) exhibiting exceptional removal efficiencies (>92%) toward short-chain PFAAs at environment-relevant concentrations. Besides, according to Sörengård et al (2020) [73] that studied on adsorption behavior of PFASs to 44 inorganic and organic sorbents, it has shown that magnesium chloride-fortified-biochar was one of the best sorbents. The results of Zhang et al. (2019) [42] indicated that adsorption technology is a feasible method to control the contamination of PFAS, and both GAC and BC are effective adsorbents for PFASs removal from wastewater. However, GAC is most effective for long-chain PFAS. Guo et al (2017) [74] investigated the adsorption behavior of PFOS on BC produced from corn straw at varying temperatures. They found high pyrolytic temperature resulted in the increase of pore structure, larger surface area, and better adsorption capacity of PFOS. At this year, Inyang and Dickenson (2017) [75] also explored that hardwood and pinewood-derived biochar achieved high PFOA removal performance.
Regarding the using of nanomaterials in remediation, in the study of Pan and Xing (2008) [76], carbon nanotubes (CNTs) have been investigated as a potential candidate for the removal of PFASs from water, owing to the intense adsorption affinity between CNTs and organic pollutants.
Combination of different biological techniques
To eliminate the level of PFAS pollution in the environment, appropriate treatment technologies should be used in both water and soil. In fact, a combination of different remediation strategies may be needed to achieve this goal [60].
All bacterial, algal and fungal species are effective in the degradation of PFAS species. For example, Pseudomonas sp. seems to be a desirable candidate for PFAS biodegradation. The presence of competing chemicals affects biodegradation efficiency. Novel biological/combined methods are required for complete PFAS degradation [77].
Several studies have shown that the presence of plants may enhance the degradation of organic pollutants in the soil and may also change their degradation pathways since for instance, the Plant root exudates can accelerate contaminant degradation in the root zone by increasing microbial populations and activities in the rhizosphere [6]. Arslan and Al-Din (2021) also studied the performance of wetlands in the removal of poly-fluoroalkyl substances and studied the mechanisms of removal through wetland plants and the coexistent microbes in PFAS bioremediation.
The literature review of Svensson (2021) [78] revealed that the combination of biochar and phytoremediation could be useful as these two techniques targets different variations of PFAS which shows a potential for them to target a larger range of PFAS variations if they were to be applied together and should be further investigated. The case study results showed that Miscanthus and Salix can be useful to reduce the amount of leachate within the landfill, leading to lower release rates from the landfill. Furthermore, based solely on biomass production it does seem that Salix can be useful for accumulating PFAS which can then be removed from the landfill.
Furthermore, Silva et al (2022) [79] explored the impact of PFAS on different trophic groups in anaerobic communities. They also revealed the advantage of bringing Anaerobic digestion and carbon materials together for PFAS remediation. In another study, the most efficient removal was found in the reed beds with three plant species for all PFASs, where the reed bed soil retained PFOA and PFOS while the reeds accumulated short-chain PFAAs. The combination of soil sorption and plant uptake contributed 40.7–99.6% to the overall PFAS removal in the constructed wetland system [45].
Furthermore, about the combination of algae use and biosorption, algae can be introduced as an ideal and promising biosorbent. Based on various studies on biosorption, algae have been used as a biosorbent material, which is 15.3% more effective than other types of biomasses and 84.6% more than other microbial biosorbents. Overall, owing to its fast bioabsorption, low cost and high efficiency, reusability, and non-toxic waste generation, algae can be an ideal and promising biosorbent [80].
Furthermore, Based on the study of Bolan et al (2021) [38], PFAS can be removed via phytoremediation and soil washing using surfactants. They also demonstrated that bioremediation is not effective enough for the destruction of biowaste-derived PFAS. Besides, according to the study of Sharma et al (2019) [81], algal biochar reinforced trimetallic nanocomposite was successfully fabricated for wastewater remediation due to its promising adsorption/ photocatalytic potential.
There is also a case study on microalgal-assisted bacterial biodegradation. Microalgae may also play a role in enhancing bacterial biodegradation of ECs. In microalgae-bacteria coupled treatment systems, such as wastewater high-rate algal ponds (HRAPs), microalgal photosynthesis provides the necessary oxygen, a key electron acceptor, for aerobic bacterial degradation of the organic compounds, which, in turn, provides the CO2 required for microalgal photosynthesis [82].
In case of using bioremediation and phytoremediation techniques, Arslan and El-Din (2021) [83] discussed that typically, key removal processes for PFAS removal are sorption, bioaccumulation, and plant uptake that among which, the plant-microbe mechanism opens new opportunities for better removal. They also insisted that Genetic/molecular mechanisms for PFAS transformation are crucial to investigate. For instance, the plant–microbe interplay in a wetland system could reduce C–F bonds in PFOA and PFOS, leading to their mineralization.
In another study by Zhao et al. (2014) [84], it was illustrated that PFASs can be taken from the soil by wheat roots and translocated to the shoot. The biota-to-soil accumulation factor (BSAF) in earthworms increased with increasing carbon chain length and finally they showed that the coexistence of wheat and earthworm improves the bioavailability of PFAS in soil. In fact, the study of the increasing bioconcentration potential of PFASs through wheat-earthworm interactions showed that wheat and earthworm reciprocally increased the bioconcentrations of PFAAs, with the exception that the bioconcentrations of PFAS with more than seven carbon dioxides had a negative effect [84].
Limitations and future perspective
Biological remediation has several advantages over other remediation methods, including fewer chemical and physical disturbances in soil and water environments and lower capital and operating costs. However, each method can have its own limitations. For this reason, attributes, pros and cons of different biological remediation methods for PFAS contaminated environments are summarized in Table 1.
Bioremediation category | Process | Suitability | Limitations |
Microbial degradation | Using microorganisms to consume and truncate PFAS pollutants.Microbes use organic pollutants as their energy source. | Low technology/low initial cost and minimal equipment. Applies a natural process. | PFAS does not readily biodegrade. There is a possibility to truncate long chain PFAS into stable medium chain PFAS. The process may take a comparatively longer treatment and long biotransformation time [18]. |
Fungal degradation | Fungi use chemical substances as carbon and energy source for metabolism, thereby breaking down larger molecules into smaller ones. Some fungal species that are resistant to pollutants reduce the concentration of contaminants in aerial parts of the plant by preventing the transfer of contaminants from the roots to the shoots. | environmentally- friendly Applies a natural process. Some species have high ability to produce extracellular degrading enzymes and high degradation power. High efficiency to reduce some contaminants. | When using fungal enzyme to catalyze oxidation in real life, many unknown potential problems such as the fluidity of water, time, cost and reaction conditions are likely to limit the use of enzyme. |
Algal degradation | Using (macro/micro) algae to remove pollutants from the environment or to convert them into harmless form. | A sustainable and eco-friendly approach. Low requirement of nutrients, high sorption capacity, high surface area to volume ratio. Greater production biomass. | Algae biomass separation from water, process efficiency in cold climate, and the algae biomass ability to reduce micropollutants content in wastewater [86]. |
Phytoremediation | Using plants which can absorb perfluorinated compounds, causing them to break down or immobilize | Cost-saving and suitable. approach for large-scale pollution control. | Long time, the uncertainty of the toxicity of the products, and incomplete removal. |
Vermiremediation | The process of biodegradation of organic matter through the interactions between earthworms and microorganisms | An evolving environmental- friendly and sustainable technology. Some organics that otherwise cannot be utilized by others, can be utilized by this technology. This includes about 100–1000 times greater value enhancement as compared to other biological technologies. | Earthworms are applicable only to less toxic soil, and to the certain depths of the soil. Sensitive to any change in environmental conditions [87]. |
Bioadsorption | The ability of biological materials to accumulate pollutants from the environment through metabolically mediated (by the use of ATP) or spontaneous physicochemical pathways of uptake (not at the cost of ATP), or as a property of certain types of inactive, non-living microbial biomass which bind and concentrate pollutants (Shamim, 2018). | High removal efficiency, economic and environmentally- friendly, convenient and fast for field-scale. limited use of chemicals, ability to remove contaminants in wastewater even in very dilute concentrations. | Longer chains PFASs have higher removal efficiency. pollutants are not destroyed but simply transferred. bioabsorption capacity can decrease after several regeneration cycles. still more common at the laboratory scale. Several biosorbents have not been commercially successful in a sustained context. Still limited understanding of the complex and interrelated mechanisms involved [88]. |
Some researchers have shown an increase in stable PFAS, after biological processes, possibly by biodegradation of precursors such as fluorotelomer sulfonates and alcohols to stable PFAS. PFAS bioremediation could be advanced with identification of more candidate species for bioremediation, optimization of bioremediation conditions, mixed culturing, experiments with environmental media, and studies on the biochemical pathways of biotransformation. Preventing secondary pollution is of grate importance that must be considered. It is also worth to be noted that, meta-analysis is one of the best approaches for future studies to understand the effectiveness of combined methods.
While several methods have been developed to remove PFAS from the environment, including adsorption, filtration, thermal treatment, chemical oxidation/reduction, and soil washing, these technologies are expensive, impractical for in situ treatment, and apply high pressures and temperatures, with most resulting in toxic waste. Through the application of new technologies in microbial ecology, such as stable isotope probing, metagenomics, transcriptomics, and metabolomics, there is the potential to examine and identify the biodegradation of PFAS [85]. In conclusion, different biological methods of removing or reducing PFAS from the environment were investigated and each method has disadvantages and limitations. There are several individuals and combined methods under investigation. The feasibility of these methods should be verified by following laboratory and field applications as there has been no biological technique that can completely remove PFAS at present. However, its removal techniques are developing continuously.
Hybrid techniques are more effective, energy efficient than single technique alone. The unique physicochemical properties of different PFASs impose difficult challenges. Therefore, careful selection of an effective hybrid treatment method in an integrated processing unit would be a revolutionary approach to the complete removal of PFASs from the environment.
References
- Perfluoroalkyl and polyfluoroalkyl substances in the environment: terminology, classification, and origins Buck RC , Franklin J, Berger U, Conder JM , Cousins IT , Voogt P, Jensen AA , Kannan K, Mabury SA , Leeuwen SPJ . Integrated Environmental Assessment and Management.2011;7(4). CrossRef
- Degradation of Perfluorosurfactant in Aqueous Solution using Non-thermal Plasma Generated by Nano-second pulse Corona Discharge Reactor Hama Aziz K, Miessner H, Mahyar A, Müller S, Möller D, Saeed F, Omer K. Arabian Journal of Chemistry.2021;14. CrossRef
- Guideline levels for PFOA and PFOS in drinking water: the role of scientific uncertainty, risk assessment decisions, and social factors Cordner A, De La Rosa VY , Schaider LA , Rudel RA , Richter L, Brown P. Journal of Exposure Science & Environmental Epidemiology.2019;29(2). CrossRef
- A Never-Ending Story of Per- and Polyfluoroalkyl Substances (PFASs)? Wang Z, DeWitt JC , Higgins CP , Cousins IT . Environmental Science & Technology.2017;51(5). CrossRef
- Serum perfluorooctanoic acid and perfluorooctane sulfonate concentrations in relation to birth outcomes in the Mid-Ohio Valley, 2005-2010 Darrow LA , Stein CR , Steenland K. Environmental Health Perspectives.2013;121(10). CrossRef
- Associations between Polyfluoroalkyl Substances Exposure and Breast Cancer: A Meta-Analysis Jiang H, Liu H, Liu G, Yu J, Liu N, Jin Y, Bi Y, Wang H. Toxics.2022;10(6). CrossRef
- Gingerol/letrozole-loaded mesoporous silica nanoparticles for breast cancer therapy: In-silico and in-vitro studies Akbarzadeh I, Poor AS . Microporous and Mesoporous Materials.2022;337:111919.
- Niosomes: a novel targeted drug delivery system for cancer Moghtaderi M, Sedaghatnia K, Bourbour M, Fatemizadeh M, Salehi Moghaddam Z, Hejabi F, Heidari F, Quazi S, Farasati Far B. Medical Oncology (Northwood, London, England).2022;39(12). CrossRef
- Preparation, Optimization and In-Vitro Evaluation of Curcumin-Loaded Niosome@calcium Alginate Nanocarrier as a New Approach for Breast Cancer Treatment Akbarzadeh I, Shayan M, Bourbour M, Moghtaderi M, Noorbazargan H, Eshrati Yeganeh F, Saffar S, Tahriri M. Biology.2021;10(3). CrossRef
- Effects of Cisplatin-Loaded Niosomal Nanoparticleson BT-20 Human Breast Carcinoma Cells Kanaani L, Javadi I, Ebrahimifar M, Ebrahimi Shahmabadi H, Akbarzadeh Khiyav A, Mehrdiba T. Asian Pacific journal of cancer prevention: APJCP.2017;18(2). CrossRef
- PFAS and cancer, a scoping review of the epidemiologic evidence Steenland K, Winquist A. Environmental Research.2021;194. CrossRef
- The Sources of Essential Fatty Acids for Allergic and Cancer Patients; a Connection with Insight into Mammalian Target of Rapamycin: A Narrative Review Arshad Z, Rezapour-Firouzi S, Mohammadian M, Ebrahimifar n. Asian Pacific journal of cancer prevention: APJCP.2018;19(9). CrossRef
- Engineering and characterization of dehalogenase enzymes from Delftia acidovorans in bioremediation of perfluorinated compounds Harris JD , Coon CM , Doherty ME , McHugh EA , Warner MC , Walters CL , Orahood OM , et al . Synthetic and Systems Biotechnology.2022;7(2). CrossRef
- Emerging investigator series: a 14-year depositional ice record of perfluoroalkyl substances in the High Arctic MacInnis JJ , French K, Muir DCG , Spencer C, Criscitiello A, De Silva AO , Young CJ . Environmental Science. Processes & Impacts.2017;19(1). CrossRef
- A review of emerging technologies for remediation of PFASs Ross I, McDonough J, Miles J, Storch P, Thelakkat Kochunarayanan P, Kalve E, Hurst J, S. Dasgupta S, Burdick J. Remediation Journal.2018;28(2). CrossRef
- Occurrence and fate of perfluorochemicals in soil following the land application of municipal biosolids Sepulvado JG , Blaine AC , Hundal LS , Higgins CP . Environmental Science & Technology.2011;45(19). CrossRef
- Opinion of the scientific panel on contaminants in the food chain on perfluorooctane sulfonate (PFOS) Benford D, De Boer J, Carere A, Di Domenico A, Johansson N, Schrenk D, Dellatte E. perfluorooctanoic acid (PFOA) and their salts.2008;EFSA J(653):1-131.
- Research progress on the removal of hazardous perfluorochemicals: A review Li P, Zhi D, Zhang X, Zhu H, Li Z, Peng Y, He Y, Luo L, Rong X, Zhou Y. Journal of Environmental Management.2019;250. CrossRef
- Microbial Enzymes Based Technologies for Bioremediation of Pollutions Behbudi G, Yousefi K, Sadeghipour Y. 2021;9. CrossRef
- Phytoremediation of Soil Contaminated with Petroleum Hydrocarbons: A Review of Recent Literature Kuok Ho DT . Global Journal of Environmental Science and Management.2019;1.
- Phytoremediation potential for poly-and perfluoroalkyl substances (PFASs) using various plant species Wu TC . 2021.
- Efficacy of Vermiremediation to Remove Contaminants from Soil Dada EO , Akinola MO , Owa SO , Dedeke GA , Aladesida AA , Owagboriaye FO , Oludipe EO . Journal of Health & Pollution.2021;11(29). CrossRef
- Vermiremediation of agrochemicals. In Agrochemicals Detection, Treatment and Remediation Usmani Z, Rani R, Gupta P, Prasad MNV . Butterworth-Heinemann..;:329-367.
- Recent advances in remediation of synthetic dyes from wastewaters using sustainable and low-cost adsorbents Siddiqui SI, Fatima B, Tara N, Rathi G, Chaudhry SA . The impact and prospects of green chemistry for textile technology.;:471-507.
- Soil bioremediation by cyclodextrins. A review Morillo E., Madrid F., Lara-Moreno A., Villaverde J.. International Journal of Pharmaceutics.2020;591. CrossRef
- Remediation of water from per-/poly-fluoroalkyl substances (PFAS)-challenges and perspectives Garg S, Wang J, Kumar P, Mishra V, Arafat H, Sharma RS , Dumée LF . Journal of Environmental Chemical Engineering.2021;:105784.
- Can Microbes Save Us from PFAS? Lim X. ACS Central Science.2021;7(1). CrossRef
- Microbial Defluorination of Unsaturated Per- and Polyfluorinated Carboxylic Acids under Anaerobic and Aerobic Conditions: A Structure Specificity Study Yu Y, Che S, Ren C, Jin B, Tian Z, Liu J, Men Y. Environmental Science & Technology.2022;56(8). CrossRef
- Bioremediation of perfluorochemicals: current state and the way forward Tang KHD , Kristanti RA . Bioprocess and Biosystems Engineering.2022;45(7). CrossRef
- Comparative characterization of microbial communities that inhabit PFAS-rich contaminated sites: A case-control study Senevirathna STMLD , Krishna KCB , Mahinroosta R, Sathasivan A. Journal of Hazardous Materials.2022;423(Pt A). CrossRef
- Bioremediation of Per-and Poly-Fluoroalkyl Substances (PFAS) by Synechocystis sp. PCC 6803: A Chassis for a Synthetic Biology Approach. Marchetto F, Roverso M, Righetti D, Bogialli S, Filippini F, Bergantino E, Sforza E. Life.2021;11(2):1300.
- Biodegradation of PFOA in microbial electrolysis cells by Acidimicrobiaceae sp. strain A6 Ruiz-Urigüen M, Shuai W, Huang S, Jaffé PR . Chemosphere.2022;292. CrossRef
- Anaerobic degradation of perfluorooctanoic acid (PFOA) in biosolids by Acidimicrobium sp. strain A6 Huang S, Sima M, Long Y, Messenger C, Jaffé PR . Journal of Hazardous Materials.2022;424(Pt D). CrossRef
- Defluorination of Perfluorooctanoic Acid (PFOA) and Perfluorooctane Sulfonate (PFOS) by Acidimicrobium sp. Strain A6 Huang S, Jaffé PR . Environmental Science & Technology.2019;53(19). CrossRef
- Fungal Biotransformation of Polyfluoroalkyl Substances: Identification of Growth Substrates for Favorable Biotransformation Pathways (Doctoral dissertation, UCLA) Merino NSL . 2016.
- Fungal biotransformation of 6: 2 fluorotelomer alcohol. Merino N, Wang M, Ambrocio R, Mak K, Mahendra S, Gao A, O'Connor E. Remediation Journal.2018;28(2):59-70.
- Evaluation of an Algal Turf Scrubber™(ATS) Approach for the Remediation of Perfluoroalkyl Substances (PFAS) Lopes Viticoski R. 2019.
- Distribution, behaviour, bioavailability and remediation of poly- and per-fluoroalkyl substances (PFAS) in solid biowastes and biowaste-treated soil Bolan N, Sarkar B, Vithanage M, Singh G, Tsang DCW , Mukhopadhyay R, Ramadass K, et al . Environment International.2021;155. CrossRef
- Uptake, accumulation and metabolism of PFASs in plants and health perspectives: A critical review Jiao X, Shi Q, Gan J. Critical Reviews in Environmental Science and Technology.2021;51(23). CrossRef
- Uptake mechanisms of perfluoroalkyl acids with different carbon chain lengths (C2-C8) by wheat (Triticum acstivnm L.) Zhang L, Sun H, Wang Q, Chen H, Yao Y, Zhao Z, Alder AC . The Science of the Total Environment.2019;654. CrossRef
- Uptake and translocation of perfluoroalkyl acids (PFAA) in red chicory (Cichorium intybus L.) under various treatments with pre-contaminated soil and irrigation water Gredelj A, Nicoletto C, Valsecchi S, Ferrario C, Polesello S, Lava R, Zanon F, Barausse A, Palmeri L, Guidolin L, Bonato M. The Science of the Total Environment.2020;708. CrossRef
- Distribution of eight perfluoroalkyl acids in plant-soil-water systems and their effect on the soil microbial community Zhang D, Zhang W, Liang Y. The Science of the Total Environment.2019;697. CrossRef
- Plant Uptake of Per- and Polyfluoroalkyl Substances at a Contaminated Fire Training Facility to Evaluate the Phytoremediation Potential of Various Plant Species Gobelius L, Lewis J, Ahrens L. Environmental Science & Technology.2017;51(21). CrossRef
- Metabolism of Ibuprofen by Phragmites australis: Uptake and Phytodegradation He Y, Langenhoff AAM , Sutton NB , Rijnaarts HHM , Blokland MH , Chen F, Huber C, Schröder P. Environmental Science & Technology.2017;51(8). CrossRef
- Uptake, phytovolatilization, and interconversion of 2,4-dibromophenol and 2,4-dibromoanisole in rice plants Zhang Q, Kong W, Wei L, Wang Y, Luo Y, Wang P, Liu J, Schnoor JL , Jiang G. Environment International.2020;142. CrossRef
- Remediation of poly- and perfluoroalkyl substances (PFAS) contaminated soils — To mobilize or to immobilize or to degrade? Bolan N, Sarkar B, Yan Y, Li Q, Wijesekara H, Kannan K, Tsang DCW , et al . Journal of hazardous materials.2021;401. CrossRef
- Phytoremediation of poly- and perfluoroalkyl substances: A review on aquatic plants, influencing factors, and phytotoxicity Huang D, Xiao R, Du L, Zhang G, Yin L, Deng R, Wang G. Journal of Hazardous Materials.2021;418. CrossRef
- Accumulation of six PFAS compounds by woody and herbaceous plants: potential for phytoextraction Huff DK , Morris LA , Sutter L, Costanza J, Pennell KD . International Journal of Phytoremediation.2020;22(14). CrossRef
- Exposure of Juncus effusus to seven perfluoroalkyl acids: Uptake, accumulation and phytotoxicity Zhang W, Zhang D, Zagorevski DV , Liang Y. Chemosphere.2019;233. CrossRef
- Perfluoroalkyl and polyfluoroalkyl substances removal in a full-scale tropical constructed wetland system treating landfill leachate Yin T, Chen H, Reinhard M, Yi X, He Y, Gin KY . Water Research.2017;125. CrossRef
- Removal of perfluoalkyl acids (PFAAs) through fluorochemical industrial and domestic wastewater treatment plants and bioaccumulation in aquatic plants in river and artificial wetland Wang P, Zhang M, Lu Y, Meng J, Li Q, Lu X. Environment International.2019;129. CrossRef
- Bioaccumulation and biomonitoring Wang WX. In Marine Ecotoxicology .Academic Press..2016;:99-119.
- Accumulation potentials of perfluoroalkyl carboxylic acids (PFCAs) and perfluoroalkyl sulfonic acids (PFSAs) in maize (Zea mays) Krippner J, Falk S, Brunn H, Georgii S, Schubert S, Stahl T. Journal of Agricultural and Food Chemistry.2015;63(14). CrossRef
- Field study on the uptake and translocation of perfluoroalkyl acids (PFAAs) by wheat (Triticum aestivum L.) grown in biosolids-amended soils Wen B, Li L, Zhang H, Ma Y, Shan X, Zhang S. Environmental Pollution (Barking, Essex: 1987).2014;184. CrossRef
- Phytoextraction of Lead: Its Feasibility, Constraints and Concerns Kuok Ho DT . Asian Soil Research Journal.2021;5. CrossRef
- Quantitative relationship between earthworms' sensitivity to organic pollutants and the contaminants' degradation in soil: A meta-analysis Chao H, Sun M, Wu Y, Xia R, Yuan S, Hu F. Journal of Hazardous Materials.2022;429. CrossRef
- Perfluoroalkylsulfonic and carboxylic acids in earthworms (Eisenia fetida): Accumulation and effects results from spiked soils at PFAS concentrations bracketing environmental relevance Karnjanapiboonwong A, Deb SK , Subbiah S, Wang D, Anderson TA . Chemosphere.2018;199. CrossRef
- Ecotoxicological effects of per- and polyfluoroalkyl substances (PFAS) and of a new PFAS adsorbing organoclay to immobilize PFAS in soils on earthworms and plants Melo TM , Schauerte M, Bluhm A, Slaný M, Paller M, Bolan N, Bosch J, Fritzsche A, Rinklebe J. Journal of Hazardous Materials.2022;433. CrossRef
- Microbial and plant derived biomass for removal of heavy metals from wastewater Ahluwalia SS , Goyal D. Bioresource Technology.2007;98(12). CrossRef
- A review of the emerging treatment technologies for PFAS contaminated soils Mahinroosta R, Senevirathna L. Journal of Environmental Management.2020;255. CrossRef
- Bioremediation of Plastic Material by Using Nanotechnology. Current Advances in Mechanical Engineering: Select Proceedings of ICRAMERD 2020, 27 Priyadarsini M, Biswal T. 2021.
- Adsorption of perfluoroalkyl and polyfluoroalkyl substances (PFASs) from aqueous solution - A review Zhang DQ , Zhang WL , Liang YN . The Science of the Total Environment.2019;694. CrossRef
- Comparison of currently available PFAS remediation technologies in water: A review Wanninayake DM . Journal of Environmental Management.2021;283. CrossRef
- Biochar Assisted Remediation of Toxic Metals and Metalloids Dhiman S, Ibrahim M, Devi K, Sharma N, Kapoor N, Kaur R, Bhardwaj R. Handbook of Assisted and Amendment: Enhanced Sustainable Remediation Technology.2021;:131-162.
- Adsorption behavior of perfluorooctanesulfonate (PFOS) onto activated spent coffee grounds biochar in synthetic wastewater effluent Steigerwald JM , Ray JR . Journal of Hazardous Materials Letters.2021;2. CrossRef
- Bioremediation strategies with biochar for polychlorinated biphenyls (PCBs)-contaminated soils: A review Valizadeh S, Lee SS , Baek K, Choi YJ , Jeon B, Rhee GH , Andrew Lin K, Park Y. Environmental Research.2021;200. CrossRef
- Biochar Water Treatment for Control of Organic Micropollutants with UVA Surrogate Monitoring Kearns J, Dickenson E, Aung MT , Joseph SM , Summers SR , Knappe D. Environmental Engineering Science.2021;38(5). CrossRef
- Nano-biochar reduced soil erosion and nitrate loss in sloping fields on the Loess Plateau of China Chen X, Zhou B, Wang Q, Tao W, Lin H. Catena.2020;187:104346.
- Nanobiochar and biochar based nanocomposites: Advances and applications Chausali N, Saxena J, Prasad R. Journal of Agriculture and Food Research.2021;5. CrossRef
- Biochar sorption of perfluoroalkyl substances (PFASs) in aqueous film-forming foams-impacted groundwater: Effects of PFASs properties and groundwater chemistry Vo HNP , Nguyen TMH , Ngo HH , Guo W, Shukla P. Chemosphere.2022;286(Pt 1). CrossRef
- Removal of PFASs from biosolids using a semi-pilot scale pyrolysis reactor and the application of biosolids derived biochar for the removal of PFASs from contaminated water Kundu S, Patel S, Halder P, Patel T, Marzbali MH , Pramanik BK , Paz-Ferreiro J, et al . Environmental Science: Water Research & Technology.2021;7(3). CrossRef
- Efficient adsorptive removal of short-chain perfluoroalkyl acids using reed straw-derived biochar (RESCA) Liu N, Wu C, Lyu G, Li M. The Science of the Total Environment.2021;798. CrossRef
- Adsorption behavior of per- and polyfluoralkyl substances (PFASs) to 44 inorganic and organic sorbents and use of dyes as proxies for PFAS sorption Sörengård M, Östblom E, Köhler S, Ahrens L. Journal of Environmental Chemical Engineering.2020;8. CrossRef
- Adsorption of perfluorooctane sulfonate (PFOS) on corn straw-derived biochar prepared at different pyrolytic temperatures Guo W, Huo S, Feng J, Lu X. Journal of the Taiwan Institute of Chemical Engineers.2017;78. CrossRef
- The use of carbon adsorbents for the removal of perfluoroalkyl acids from potable reuse systems Inyang M, Dickenson ERV . Chemosphere.2017;184. CrossRef
- Adsorption mechanisms of organic chemicals on carbon nanotubes Pan B, Xing B. Environmental Science & Technology.2008;42(24). CrossRef
- Distribution of perfluoroalkyl substances (PFASs) in aquatic plant-based systems: From soil adsorption and plant uptake to effects on microbial community Zhang DQ , Wang M, He Q, Niu X, Liang Y. Environmental Pollution (Barking, Essex: 1987).2020;257. CrossRef
- The potential for binding PFAS using biochar and phytoremediation Svensson JAM . Student thesis series INES.2021.
- Bioremediation of Perfluoroalkyl Substances (PFAS) by Anaerobic Digestion: Effect of PFAS on Different Trophic Groups and Methane Production Accelerated by Carbon Materials Silva AR , Duarte MS , Alves MM , Pereira L. Molecules (Basel, Switzerland).2022;27(6). CrossRef
- Application of biosorption for removal of heavy metals from wastewater. Biosorption, 18(69), 70-116. Kanamarlapudi SLRK , Chintalpudi VK , Muddada S . 2018.
- Algal biochar reinforced trimetallic nanocomposite as adsorptional/photocatalyst for remediation of malachite green from aqueous medium Sharma G, Bhogal S, Gupta VK , Agarwal S, Kumar A, Pathania D, Stadler FJ . Journal of Molecular Liquids.2019;275:499-509.
- Microalgal bioremediation of emerging contaminants - Opportunities and challenges Sutherland DL , Ralph PJ . Water Research.2019;164. CrossRef
- Removal of per- and poly-fluoroalkyl substances (PFASs) by wetlands: Prospects on plants, microbes and the interplay Arslan M, Gamal El-Din M. The Science of the Total Environment.2021;800. CrossRef
- Mutual impacts of wheat (Triticum aestivum L.) and earthworms (Eisenia fetida) on the bioavailability of perfluoroalkyl substances (PFASs) in soil Zhao S, Fang S, Zhu L, Liu L, Liu Z, Zhang Y. Environmental Pollution (Barking, Essex: 1987).2014;184. CrossRef
- Challenges and Current Status of the Biological Treatment of PFAS-Contaminated Soils Shahsavari E, Rouch D, Khudur LS , Thomas D, Aburto-Medina A, Ball AS . Frontiers in Bioengineering and Biotechnology.2020;8. CrossRef
- USE OF ARTIFICIAL AQUATIC FOOD-WEB FOR POST-TREATMENT OF DOMESTIC WASTEWATER IN COLD CLIMATE: A REVIEW Lavrinovičs A, Juhna T. Journal of Water Security.2017;3. CrossRef
- Vermiremediation of organically contaminated soils: Concepts, current status, and future perspectives Shi Z, Liu J, Tang Z, Zhao Y, Wang C. Applied Soil Ecology.2020;147:103377.
- Biosorption of heavy metals Shamim S. Biosorption.2018;2:21-49.
Author Details
How to Cite
- Abstract viewed - 0 times
- PDF (FULL TEXT) downloaded - 0 times
- XML downloaded - 0 times