Unleashing Translational Opportunities of Loco-Regional Drug Delivery to Treat Malignancy
Download
Abstract
Background: Loco-regional drug delivery for cancer treatment has emerged as a promising approach to enhance therapeutic efficacy while minimizing systemic toxicity. Various strategies have been developed, including localized drug delivery systems and targeted delivery methods, to improve drug distribution and retention at tumor sites. Despite significant progress, several challenges persist, necessitating further exploration and innovation.
Objective: This review aims to provide insights into the recent advances and challenges in loco-regional drug delivery for cancer therapy and identify future translational opportunities in this field.
Materials and Methods: A literature search was conducted on loco-regional drug delivery systems for cancer treatment, identifying advancements in nanoparticle-based formulations, hydrogels, implants, and image-guided delivery techniques. Challenges include drug resistance, limited tumor tissue penetration, and tumor microenvironment complexity.
Conclusion: Loco-regional drug delivery has the potential to transform cancer therapy by allowing for precision targeting, combining therapeutic modalities, using nanotechnology, and utilising modern imaging tools.
Discussion: Collaboration among academics, physicians, and industry stakeholders is critical for expediting the clinical translation of innovative drug delivery platforms, which have the potential to drastically improve malignancy patient outcomes as well as enable personalised treatment methods.
Introduction
Cancer is one of the world’s most destructive diseases, and, according to the World Health Organisation, it is on course to overtake cardiovascular disease as the top cause of death worldwide, accounting for more than eight million deaths each year [1]. Systemic chemotherapy is a crucial aspect of cancer treatment. However, because anticancer medications are frequently administered at high doses, considerable dose-dependent toxicities are unavoidable.
Furthermore, insufficient tumor therapy with systemic chemotherapeutics (for example, exposing the tumor to sub-lethal drug dosages) would result in the selection of drug-resistant tumor cells, complicating successful cancer treatment even further [2].
The success or lack thereof of chemotherapy is influenced not only by the medication itself but also by how it is given to its intended target. Even under optimal conditions, chemotherapy medicines almost always cause harm to normal tissue because of their relatively nonspecific action.
Therefore, one must prepare to selectively direct the power source antineoplastic representative with the tumor target as specifically as possible to minimize the systemic adverse reactions caused by generalized systemic distribution, as well as having the ability to use a much smaller dose, which would further reduce toxicity [3].
Previously, chemotherapy based on magnetic fields and magnetic albumin microspheres produced promising results. According to 1996, a novel FF,3 which is described in detail below, was used in trials in which tumor-bearing experimental animals (bare-chested mice and rats) were injected i.v. with an FF complex (magnetic drug) that was
driven into the tumor using a magnetic field.
The animals tolerated the FF complex well and were able to achieve tumor remission. As a second stage, the first Phase I clinical trial using this approach was conducted on patients with advanced, unsuccessfully treated malignancies or sarcomas [4]. The “magnetic drug targeting” approach was widely adopted.
Cancer treatment has traditionally been based on three pillars: surgery, chemotherapy, and radiation, with immunotherapies becoming more important in particular cancers. Standard pharmaceutical regimens are often given to patients by intravenous (IV) injection, which includes concomitant off-target detrimental side effects that cause substantial patient morbidity due to a lack of therapeutic specificity [5]. Even when systemically administered chemotherapeutics reach the region of interest, penetration into the tumor mass may be difficult due to the altered tumor microenvironment (TME).
This demands high systemic doses to elicit a therapeutic response, worsening individual adverse reaction patterns.
Surgery is the preferred treatment for the vast majority of solid tumors. In many cases, however, entire excision by surgery is not possible because occult tumor nodules may go undetected, leading to cancer recurrence [6].
As a result, localized recurrence of tumors following surgery has been observed in various cancer types, including the brain, colon, and lungs.
To address the lack of specificity in standard chemotherapeutic drugs and prevent cancer recurrence after surgery, localized chemotherapy using polymeric material drug depots is a potential technique. Drug-loaded microparticles have several advantages over traditional forms of administration, including increased frequency of action to provide short-half-life drugs, bypassing various biological barriers in the body by directly conducting the drug into the area of interest (e.g., through intratumoral implantation in addition to adjacent to the tumor), and sustaining drug action over time [7].
The bulk of the cases considered in this paper include drug depots formed of polymeric microparticles, which can be injected or inhaled as is, or compacted into polymeric wafers. Figure 1 demonstrates the rationale for selecting locoregional chemotherapy over conventional treatment.
Figure 1. Demonstrates the Rationale for Selecting Locoregional Chemotherapy Over Conventional Treatment.
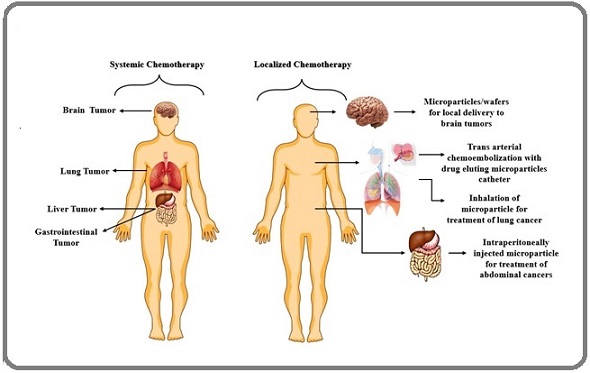
This study aims to provide a worldwide perspective on existing clinical methods for locoregional cancer therapy, as well as those under clinical investigation, with a focus on drugs in Phase II/III trials. The study begins by examining loco-regional cancer therapy based on direct pharmaceutical instillation, as well as the known data and limitations of this strategy [8].
Expanding on these limits, it investigates how drug delivery technologies have been employed to improve loco-regional drug delivery, as well as if such efforts have resulted in increased clinical acceptability of locoregional treatment or improved clinical outcomes in target tumors. The article delves into clinical trials for new technologies and applications, as well as the potential for loco-regional treatment, notably in immunotherapy [9].
Localised anticancer therapy of brain cancer with intratumorally implanted depots
The blood-brain barrier, or BBB, is a critical barrier to treating brain tumors because it prevents drugs from entering the brain parenchyma via its tightly connected cell layers, which express a range of multidrug-resistance proteins. The current treatments for malignant gliomas are surgical excision, radiation, and chemotherapy [10]. Resection is inefficient for long-term cancer care because recurrences usually occur within millimeters of the initial resection cavity, indicating that tumor cells have migrated deeply into existing brain tissue. Carmustine is a prominent treatment for brain cancer.
In contrast, when injected intravenously, carmustine has a circulation half-life of only 12 minutes and causes systemic injury to nontarget organs. Langer and Brem spent the early 1980s researching carmustine dispensing on a local scale. They developed Gliadel® wafers, which are drug depots made from a recyclable polymer poly [1,3-bis(carboxyphenoxy) propane-co-sebacic-acid (PCPP-SA) loaded with carmustine (20:80) [11]. The drug was first spray-dried into PCPP-SA microspheres, which were subsequently compressed into wafers to fill the
resection cavity.
Gliadel® wafers generated carmustine in vitro (buffer 37°C) and ex vivo (rat brain) during 7 days were investigated to assess the degradation of radiolabeled Gliadel® wafers within rabbit brains. In around 7 days, 74% of carmustine and 80% of SA were released from the polymer matrix, leaving behind a polymeric water-insoluble 1,3-bis(carboxyphenoxy) propane [s2] (CPP) copolymer. After a 9-day lag time, the CPP copolymer began to degrade, and by three weeks, about 60% of the CPP had been eliminated from the implant site [12].
It was postulated that the anhydride linkages in the more hydrophilic Sa-containing blocks degrade quicker than those in the blocks containing more hydrophilic CPP diacids. It was also shown that PCPP-SA was destroyed in vivo after 6 to 8 weeks.
Following surgical removal of the primary brain tumor, about eight Gliadel® wafers can be put into the patient’s brain via the resection cavity. In a clinical trial, Gliadel® wafers (together with surgery and, in some cases, radiation) lowered the mortality risk by 29% in patients (n = 240) with high-grade glioblastoma multiforme (GBM), the most aggressive kind of brain cancer [13]. Patients treated with Gliadel® had a median survival of 13.8 months, compared to 11.6 months in the placebo group.
The overall survival advantage over the placebo persisted in the first (59% vs 49%), second (16% vs 8%), and third (9 vs 2%) years, becoming statistically significant in the third year. After 56 months of follow-up, just two patients in the Gliadel® treatment group survived. In a recent study of rats implanted with intracranial 9L gliosarcoma, local delivery of paclitaxel using polylactofate (a copolymer of polylactide and a phosphodiester) microspheres in conjunction with radiotherapy demonstrated a synergistic improvement in survival compared to various treatments, including radiotherapy alone, paclitaxel-loaded microspheres alone, and radiotherapy accompanied by treatment with paclitaxel-loaded microspheres.
It was discovered that pretreatment with locally injected paclitaxel-loaded microspheres acts as a radiosensitizer for treating malignant gliomas [14].
According to multiple studies, the platelet-derived growth factor receptor (PDGFR) plays a crucial role in the angiogenesis of malignant gliomas, and blocking PDGFR stops tumor development. Imatinib mesylate (Gleevec®) is a molecularly targeted medicine that inhibits the action of PDGFR and has been approved by the FDA to treat chronic myeloid leukemia and gastrointestinal stromal tumors [15]. Imatinib-loaded poly(lactide-co-glycolide) (PLGA) microspheres were developed to test the viability of imatinib’s local release formulation for brain cancer treatment. PLGA is a prominent biodegradable polymer for the development of sustained-release drug depots due to its biodegradability and biocompatibility.
Imatinib-loaded PLGA microspheres were tested in human glioblastoma xenografts, both subcutaneous and orthotopic, as well as syngeneic mice cancer models. Five days after tumor inoculation, a single dose of microspheres containing 1.25 mg imatinib was injected into two areas of the tumor [16]. Local administration of imatinib microspheres resulted in a significant reduction in tumor size: 88% and 79% in s.c. human (U87-MG) and murine (GL261) glioma tumors, respectively.
Aside from s.c. cancer models, intracranial therapy with drug microspheres resulted in a 79% reduction in tumor development (human U87-MG cells injected in mice) 14 days later. Furthermore, no toxicity symptoms were seen in retrieved organs (liver, kidney, brain, heart, muscle, and spleen) after PLGA-imatinib microsphere administration [17]. In contrast to a previous study that explored imatinib’s systemic efficacy in glioma models, a significantly lower total dosage (1.25 mg single dose after local injection versus 30 recurrent doses of about 1.5 mg following systemic treatment) resulted in equivalent antitumoural effects.
These data indicate that local drug delivery can lessen systemic toxicity.
Carmustine encapsulated inside a monolithic implant within rat brains had a small diffusion distance (<0.3 mm) and required draining out of the implantation area before it could diffuse over a significant distance [18]. This was attributable to the drug’s significant transvascular permeability when in distribution.
The study discovered that medication dispersion was limited to a 3-mm radius from the insertion point. To compensate for the rapid diffusion of pharmaceutical molecules away from the injection site, drug-loaded microparticles can be injected many times to keep the therapeutic dose of the medication inside the resection margin [19].
Current clinical applications of locoregional drug delivery in cancer
The concept that loco-regional distribution of chemotherapy may be advantageous in cancer treatment is not novel; in fact, the use of numerous loco-regional chemotherapeutics has been a well-established method for over 60 years in a limited range of cancer types. These may be seen in two ways: first, in the delivery of basic chemotherapy drug solution(s), and second, in systems that attempt to optimize delivery by mixing chemotherapy drugs with delivery platforms [20].
Locally given nanomedicines to treat lung cancer
Every year, thousands of people die from lung cancer, making it one of the most critical diseases confronting modern medicine. Because of the high recurrence incidence, the current treatment consists of surgical resection followed by chemotherapy and radiation.
Some of the limitations of conventional chemotherapy techniques include unreasonable levels of systemic toxicity and insufficient drug accumulation at the tumor site [21]. The local distribution of nanocarriers containing anticancer medications has the potential to significantly improve efficacy while lowering systemic toxicity.
Local medication administration at the tumor site using nanoparticulated drug delivery devices can reduce the systemic toxicity associated with anticancer drugs administered intravenously. Furthermore, this method enables the long-term administration of an anticancer treatment [22].
Aerosol-based chemotherapy
Inhaling drugs is a promising field of research for lung disease treatment. Loco-regional deposition of pharmaceuticals in the lung by inhalation has the potential to increase and sustain drug concentrations at the disease site. One key advantage of inhalation administration for lung cancer treatment is that it allows for greater drug accumulation in the tumor. This effect can be enhanced by encapsulating the drugs in nanoparticles, which improve solubility, retention time, and cellular absorption at the desired site of action [23].
Nonetheless, transporting nanoparticles to the lungs involves numerous important challenges, including physiological and pathological characteristics that may restrict the efficacy of this method of delivery. It has been established that aerosols must have an optimum aerodynamic particle size for effective deposition in the deep lung [24]. If the particle size exceeds 5mm, the tiny particles tend to accumulate in the upper airways, including the main conducting airways, such as the larger bronchi, as well as the throat and nasal cavities, where they may be swiftly eliminated.
Particles smaller than 1mm settle slowly and can be evacuated before settling in the lungs. To achieve considerable deep-lung deposition, particles should have an aerodynamic diameter of 1mm to 5mm. Figure 2.
Figure 2. Co-morbidities Such as Asthma, Chronic Inflammation, and Cystic Fibrosis may Further Affect Airway Conduction .
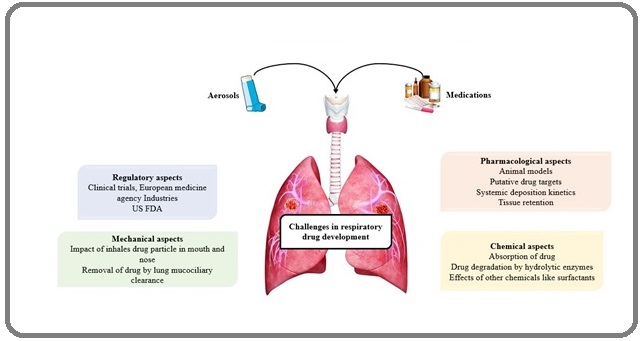
Co-morbidities such as asthma, chronic inflammation, and cystic fibrosis may further affect airway conduction. As a result of lung remodeling, such conditions can change the angles of bifurcation in the lung, the amount of mucous produced, and the rate of mucociliary clearance, all of which impact deposition in the lung and accumulation in the tumor [25].
Another issue limiting inhaled delivery is tumor size. It is widely acknowledged that a tumor bigger than 5 cm would greatly reduce the efficacy of the inhaled chemotherapeutic agent due to limited access to the 2882G cancer site and changes in airflow patterns towards the tumor. Finally, some people have voiced concerns regarding the safety of nanoparticles, especially when they are taken via the pulmonary route.
However, this is most likely due to findings from epidemiological studies including exhaled pollution nanoparticles undertaken by many groups, rather than research focussing specifically on inhaled nanomedicines. In this regard, Dailey and co-workers compared two inhaled biodegradable polymer-based tiny particle systems: the power source novel dimethylaminopropylaminopropylamine polyvinylalcohol-grafted poly(lactic-co-glycolic acid) nanoparticles (DEAPA-PVAL-g-PLGA NP) along with poly(lactic-co-glycolic acid) nanoparticles (PLGA NP), to non-biodegradable poly-styrene-based nanoparticles (PS NP) of the power source same size [26].
It was revealed that biodegradable systems resulted in decreased toxicity and inflammation in mice’s lungs following intratracheal injection. Animals treated with PS NP had significantly higher levels of lactate dehydrogenase (a marker of cellular damage) and polymorphonucleocyte recruitment, whereas no significant difference was observed between the biodegradable systems, DEAPA- PVAL-g-PLGA NP and PLGA NP, as well as the negative control, a single isotonic glucose solution.
A newly published thorough investigation of the safety of nebulised particles revealed that many of the negative effects previously described are reduced when the aerosol is disseminated optimally throughout the lung [27]. However, the opposite is frequently true when large doses produce droplet aggregation at specific locations, increasing the claimed toxicity.
This plays a crucial role in obstructed airway disorders, which can result in increased particle deposition due to changes and pauses in airflow in specific lung locations, or when nanomaterials agglomerate. Carbon nanotubes, for example, are promising drug delivery systems for a variety of applications, but they tend to aggregate and cause localized damage to airway epithelial monolayers due to altered apoptotic and proliferative rates of epithelial cells close to those aggregates.
There are four clinically useful pulmonary aerosolising devices: nebulisers, pressurised metered dosage inhalers (pMDIs), dry powder inhalers (DPIs), and soft mist inhalers. Nebulisers use an external power supply to produce liquid aerosols from a few millilitres of solutions or suspensions [28]. Air jet nebulisers use compressed gas flow, ultrasonic nebulisers use piezoelectric crystals vibrating at high frequency, and vibrating mesh nebulisers use an oscillating perforated membrane. pMDIs use liquefied gases (hydroflouroalkanes) as propellants and are portable and handy for patients.
DPIs are breath-actuated devices that use the patient’s inhaling force to disperse microparticles into an inhalable aerosol. SMIs are patient-independent devices that mechanically manufacture an aerosol by delivering a few microlitres of drug solution between two nozzles capable of forming converging jets of solution that collide, producing a thin aerosol of inhalable droplets.
Several studies have shown that the technique used for aerosolization, as well as the device properties, are critical for nanoparticle dispersal.The delivered percentage of the dose and the stage of aggregation of the particles deposited in the lung are heavily influenced by the physicochemical properties of the nanoparticles as well as the tension acting on the material to produce the aerosol throughout the device [29].
Furthermore, the efficacy of the method of delivery must be assessed in terms of potential toxicity to healthcare staff or family members around at the time of dosing. This appears to be a distinct risk, especially with nebulized formulations and will be discussed further in the next section.
Given their moderate potencies, the dose necessary to treat a lung cancer patient via inhalation might be in the milligram range. As a result, this section of the study will focus on the description of chemotherapy nanoformulations that may be delivered via nebulizers or DPI, as these methods allow for the delivery of such high doses of medication [30].
Although anticancer drugs have been formulated in metered dose inhalers (MDIs), there have been no reports in the literature of pMDIs being used to deliver anticancer drug-loaded nanomaterials.
PMDIs have been rarely used in nanoparticle formulations due to technical difficulties such as using a liquefied hydrofluoroalkane gas as a dispersant instead of water, as well as determining the aggregation status of nanoparticles within the pressurized canister and during the aerosolization phase [31].
Nebulizers
Nebulisers are among the most often used tools for creating aerosols with nanoparticle compositions. Nanosystems for lung cancer therapy are water dispersions and nebulisers that can produce nanoparticle-loaded aerosols with droplets smaller than 5mm. The viscosity, surface tension, pH, ionic strength, and osmolarity of the nanoparticle formulation will all have an impact on the aerosol created; nonetheless, apart from these alterations, the formulation will be used directly in these devices without transformation [32].
Furthermore, huge quantities of formulation may be delivered with a device that needs little or no patient coordination. Jet and ultrasonic nebulisers are the most widely available devices, and they have long been utilised in therapeutic settings. Despite their reliability and ease of administration, these devices have certain restrictions that apply to their usage in chemotherapy. Nebulised aerosol is constantly produced, and it might take several minutes for the individual undergoing treatment to inhale the appropriate quantity through a mouthpiece or facemask [33].
Without a doubt, the patient’s exhalation during tidal breathing will render at least half of the aerosol inaccessible. Typically, only approximately 10% of the dose is deposited in the lung, with the rest being distributed throughout the upper airways or lost to the environment. Furthermore, not all of the formulation is aerosolised; a residual portion remains in the device upon administration, necessitating proper waste disposal and device cleaning [34]. These latter features can pose a significant occupational risk to healthcare workers and their families.
Some new nebuliser devices, however, provide some fascinating solutions to these questions. In recent years, new inlets for aerosol inhalation, as well as breath- controlled valves, have been introduced to the market, whereas several nebuliser manufacturers (such as Pari and Aerogen) have released vibrating meshtechnology devices that have significantly reduced the time required to generate and deposit a given volume of formulation, thereby reducing drug losses due to patient exhalation [35].
However, some of these devices have recently evolved into computer-controlled systems capable of monitoring each patient’s breathing pattern and only giving aerosol in unison with inspiration (I-neb, Adaptive Aerosol Delivery System, Philips). SMIs overcome this issue by being triggered or breath actuated, using a small amount of aqueous formulation, and needing just a brief duration of nebulisation. In this way, just what the patient can inhale is delivered with each breath, and almost no medication is wasted as an aerosol to the surroundings.
Nanocarrier formulations are given as liquid aerosols
Liposomes are the most researched nanocarriers for lung delivery. Liposomes are spherical closed lipid vesicles made up of phospholipids in water. Phospholipids self-organize into one or more concentric bilayers separated by an aqueous phase. Liposomes are intriguing drug delivery vehicles due to their unusual structure. Furthermore, because phospholipids are naturally occurring molecules and a key component of alveolar surfactants, they appear to be particularly well-suited for pulmonary administration. Furthermore, non-concentric structures can be obtained when numerous unilamellar vesicles develop on the surface of larger vesicles, resulting in multivesicular vesicles (MVVs) [36].
Traditionally, liposomal formulations for inhalation have been provided by nebulisers; however, recent research have demonstrated that liposome formulations may be administered in powder form with DPIs, indicating the adaptability of this drug delivery technology.
In fact, liposomes can be designed to allow for continuous release preparation, reducing the frequency of administration and enhancing patient compliance. Surface modification of liposomes with polyethylene glycol (PEG) has been found to enhance the elimination half-life of pharmaceuticals by reducing drug absorption by alveolar macrophages, hence increasing the medications’ residence duration at the site of action. Surface modification can also be employed to promote bioadhesion or molecularly target the vesicle to cancer cells via antibody or ligand recognition [37].
Dilauroylphosphatidylcholine (DLPC) liposomes were loaded with 9-nitrocamptothecin (9NC-DLPC) by freeze- drying a butanol/DMSO solution containing the drug and phospholipids, followed by extemporaneous re-dispersion with water for injection. When the liposomal formulation was aerosolized with an air jet nebulizer (Aerotech II, CIS- US, Bedford, MA), the particle size ranged from 100-400 nm, drug encapsulation was greater than 80%, and the mass median aerodynamic diameter (MMAD) was 1.2mm. Preliminary experiments were conducted in mice using the subcutaneous human lung tumor xenograft and human osteosarcoma pulmonary metastatic models. The first trial revealed a substantial reduction in tumor volume in animals treated with aerosolized 9NC-DLPC, when compared to groups of animals given the same dosage orally or intramuscularly.
In the metastases model, the aerosolized formulation of the camptothecin derivative demonstrated the ability to lower the number of animals with illness, as well as the number and size of lung nodules in the percentage of animals with pulmonary metastases [38].
A phase I clinical study using the suggested formulation was conducted in individuals with primary or metastatic lung illnesses who did not respond to traditional therapies. Six patients were enrolled and given 6.7mg 9NC/kg by aerosolization every day for five days in a row every three weeks if their condition remained stable. This feasibility research demonstrated that aerosol delivery of liposomal 9-NC was well tolerated, with no adverse effects more than grade 2, and that 9-NC was absorbed systemically, with a plasmatic concentration peak 2 hours after administration.
Two individuals had their disease stabilized.
Inhalable SPIONs targeting the EGFR were used to achieve targeted hyperthermia for lung cancer. EGFR- targeted SPIO nanoparticles (about 370 nm) were synthesized by adding ammonium hydroxide to ferric and ferrous chloride and then coated with myristic acid and pluronic F127 conjugated with an EGFR-targeting peptide. When put in an alternating magnetic field (6 kA/m, 386 kHz), nanoparticle dispersions heated rapidly [39].
The nanoparticles were administered to mice carrying an orthotopic lung cancer model using an ultrasonic atomizer (MMAD 1.1mm), and EGFR targeting induced enhanced tumour retention and resulted in a significant reduction of lung tumor growth overcontrols, i.e. animals that were not treated, treated with non-targeted nanoparticles, or treated with targeted or non-targeted SPIONs but not subjected to magnetic hyperthermia.
While there have been some successful trials of nebulized nanoparticles for inhalation, there are certain potential issues that must be addressed before any of these products become accessible. Some major issues encountered include the potential for nanoparticles to agglomerate in suspension, poor drug stability due to hydrolysis and degradation, the potential to induce bronchoconstriction, poor deposition due to shallow tidal breathing, [40] and risks to those administering the dose.
Dry powder inhalers
DPIs may be able to address some of the concerns concerning anticancer drug delivery by nebulization, particularly those related to poor stability and risks to patients, carers, and the environment through aerosol emission. However, nanosized particles have a lower lung deposition rate and are more likely to be expelled. Furthermore, because of their size and high specific surface, nanoparticles have a high surface energy, resulting in non-dispersible clumps when dried. Nanoparticles must be delivered following integration into bigger structures of adequate size for pulmonary medication delivery [41]. A few different ways have been used to temporarily shift nanoparticles to micron-sized particles, allowing for more efficient dosing, aerosolization, and deposition of the powder in the lung. Most crucial, once deposited on the pulmonary mucosa, nanoparticles should return to their original size and execute their therapeutic function. Spray-dried powders incorporating nanoparticles and water-soluble excipients are the most established and investigated technique.
Spray drying techniques have various benefits, including the comparatively simple generation of particles with a restricted size range and the required characteristics for pulmonary delivery. This overcomes the limits associated with alternative drying processes, such as freeze-drying: the creation of hard cakes, the requirement for additional micronization, the necessity for large and/ or heterogeneous particle size distributions, the need for coarse carriers for dosing and aerosolization, and poor aerodynamic performance [42].
Furthermore, DPIs as pulmonary delivery devices have several advantages over other technologies, including long-term formulation stability, no need for hand-lung inhalation coordination because they are generally breath-actuated inhalers, improved patient compliance, uniform deposition of a high dose of drug locally, liquid and/or propellant-free formulation, and the possibility of patent protection. The formulation approach might solve challenges such as particle aggregation by minimizing inter-particle attraction by utilizing strategies such as mixing nanoparticles with bigger carrier particles like lactose, generating ordered mixtures and therefore increasing product stability [43].
Perhaps most importantly, aerosol formation is not a concern in DPIs since it is breath-actuated, hence no aerosol is lost to the environment. As seen in the section on nebulizers, the use of liposomes in pulmonary administration offers several potential benefits, including carrier appropriateness for most lipophilic medicines, water compatibility, prolonged release, and intracellular delivery.
Although liposomal formulations for inhalation treatment have traditionally been administered by nebulization, there has been a great deal of interest in converting those nanocarriers into dry powder formulations. In reality, liposomes in aqueous dispersions undergo lipid breakdown, sedimentation, aggregation, drug leakage, or fusion during storage, whereas aerosolization may cause chemical and physical instability. Their composition as a dry powder ensures long-term stability and enables administration with DPIs [44].
Pre-treatment with liposomal encapsulated p53 resulted in increased cytotoxicity and up to a 60% increase in drug sensitivity. The dry powder formulation revealed the potential for considerable lung deposition utilizing an Anderson Cascade Impactor and Rotahaler (Cipla, India) as a model capsule-based aerosolizing device with an average aerodynamic diameter of 3-4mm and a fine particle percentage of roughly 35%.
Pactlitaxel-loaded lung surfactant mimetic PEGylatedphospholipid micro/nanoparticles were produced for lung cancer drug delivery by organic solution co-spray drying of a dilute feed methanol solution of the drug with DPPC and dipalmitoyl phosphatidylethanolamine poly(ethylene glycol) (DPPE- PEG) [45].
The powders’ aerosolization ability was tested in vitro using the high-resistance capsule-based Handihaler device (Boehringer Ingelheim, Germany) and the Next Generation Impactor (NGI). The paclitaxel/phospholipid powders had mass median aerodynamic diameters ranging from 3.4-6.8mm and 2890G. Garrastazu Pereira et al. Drug Deliv, 2016; 23(8): 2881-2896 respirable fractions ranging from 44.0 to 54.5%.
Despite liposomes’ high versatility as drug delivery devices, it appears from the available literature that, in the specific case of inhaled chemotherapy, nanoparticulate carriers have been preferred over phospholipid vesicles in the case of dry powder inhalation, possibly due to their peculiar structure, which is more sensitive to the drying process than nanoparticles. A simple technique presented is the controlled dry construction of nanoparticles with good aerosolizing capabilities [46].
Paclitaxel nanoparticles were prepared by ultrasound- controlled precipitation in the presence of lecithin, polyvinylpyrrolidone, or cetyl alcohol as stabilizers, then assembled into low-density microparticles with the amino acid leucine as a colloid destabilizer and freeze-dried. When tested directly without a DPI device using a Tisch Ambient Cascade Impactor, the powders obtained not only gave quicker dissolving rates than micronized paclitaxel but also exhibited a tiny MMAD (52mm) with a high fine particle percentage (480%).
DPIs appear to be a promising delivery system for loco- regional delivery of nano-encapsulated chemotherapeutic agents, given the benefits of increased stability and control over particle size, as well as reduced complications such as pH- and osmolarity-induced bronchoconstriction observed in nebulized formulations [47].
However, as noted in Zarogoulidis’ 2012 evaluation of inhaled treatments, a key disadvantage of dry powder therapies is that a considerable percentage of the dosage is frequently retained within the device.
Furthermore, it is typical for DPI equipment to be overused, resulting in decreased effectiveness. While methods to overcome such limitations have been investigated, such as modifying devices to accommodate different inspiratory flow rates, such as the Turbohaler, dry powder platforms are currently not ready for the administration of chemotherapy to treat lung cancer, as evidenced by the lack of clinical trials using this approach. However, once this barrier is achieved, the technique looks to have a high potential for anticancer therapy delivery in the outpatient environment.
Intratumoral injection
While inhaled treatments appear to be a potential step towards better lung cancer treatment, one key barrier to their utilization is that the efficacy of deposition inside the lung is restricted by tumor size. In circumstances when surgery is not an option for tumors bigger than 5 cm, intratumoral injection may be used as an alternative to surgical resection.
This particular loco-regional administration approach, in addition to the benefits of reducing systemic exposure, may also address the issues of poor vascularization, which is frequently observed in solid tumors and inhibits penetration of the anticancer drug, resulting in low drug concentrations within the tumor parenchyma [48].
Other important biological features of solid tumors that represent significant barriers to drug absorption in inhaled therapies include altered interstitial properties, which may include stiffening of the extracellular matrix (ECM) and an increase in interstitial pressure. Most medications can only reach the tumor’s periphery, increasing the risk of metastasis or relapse since not all malignant cells can be removed, whereas intratumoral injection delivers the treatment to the tumor’s core.
It is crucial to highlight that this changed ECM is a particular problem for nanoparticles because to their large size, especially when compared to free drug. There has been much research on the intratumoral delivery of free anticancer medicines such as docetaxel, cisplatin, and paclitaxel.
The Celikoglu group has conducted some detailed assessments of the administration of cytotoxic medications by intratumoral injection, notably via bronchoscopy, and there looks to be a significant chance to achieve even more in this therapy method. As discussed in these reviews, external beam radiation has been the mainstay of treatment for inoperable lung tumors; however, it is becoming increasingly clear that this mode of treatment is insufficient, as these patients continue to have an extremely poor prognosis [49].
In a 2010 research, p53 viral vectors were co-delivered with docetaxel to tumors in mice and resulted in an amazing and considerable reduction in tumour growth in treated animals compared to the control group, with practically total tumour suppression seen. In this study, the animals had to have biweekly intratumoral injections since the medication could not be kept within the tumor, which appears to be a key limitation of practically all investigations looking into the intratumoral injection of free drug solutions.
In this context, it appears that a variety of nanosystems can be supplied using this delivery pathway to provide a sustained delivery impact. Nonetheless, little study has been conducted especially to investigate the impact of nanoparticles in intratumoral injections [50].
Kang and colleagues pioneered sustained release intratumoral delivery with an in situ-forming gel based on doxorubicin-poly(ethylene glycol)-b-caprolactone diblock copolymer (Dox-MP), which can serve as a depot for the sustained release of doxorubicin. They were able to demonstrate a persistent release of doxorubicin over 20 days following an initial burst phase during the first two days.
The result was a 10.5fold decrease in tumor volume in an animal model compared to control groups following a single dose of Dox-MP. This reduction in tumor volume was comparable to that seen with repeated (every 5 days) free doxorubicin injections [51]. The invasive nature of intratumoral injections can be reduced by using sustained release systems, and it is possible that combining the insitugel with other nanoparticle delivery systems discussed herein has a significant potential to increase efficacy and further improve the sustained release profile, minimizing patient compliance issues and improving safety profiles [52].
Even though intratumoral injection of nanoparticles laden with chemotherapy drugs was not a popular study topic until recently, a few organizations appear to have achieved significant progress. One of these organizations studied vault nanocapsule technology for delivering cytokines, especially CCL21, for lung cancer immunotherapy. Vaults are endogenous ribonucleoprotein ovoid particles that range in size from 40 to 70 nm, making them suitable carriers for therapeutic peptides and proteins [53]. Proteins may be bundled into vault particles by fusing them with a vault-targeting domain of 162 amino acids. CCL21, an immune-boosting cytokine, binds to CCR7 receptors and attracts immune cells such as naı ̈ve and memory T cells, as well as natural killer T cells, causing non-p53-mediated apoptosis.
The same group had previously investigated the delivery of this protein in its free form via intratumoral injection and succeeded in reducing tumor size in murine lung cancer models, but discovered that very high doses and frequent administration were required to overcome the drug’s rapid clearance from the tumors following injection. They have recently took this protein to Phase I clinical trials using a CCL21 gene-modified dendritic cell, but the technology was prohibitively costly and time-consuming to produce [54].
As a consequence, they created a delivery mechanism for this cytokine that uses vault nanocapsules. They discovered that a single trans-thoracic injection of recombinantCCL21-vault nanocapsules resulted in significant tumor regression due to the recruitment of antitumor effector cells such as T lymphocytes and dendritic cells in the a murine Lewis lung carcinoma model.
Immune evasion is a significant contributor to tumor development; immune stimulants such as this might be employed in concert with other cytotoxic medicines to increase the therapeutic impact. Other recent studies have concentrated on the intratumoral injection of magnetic nanoparticles in the treatment of lung cancer. One group created an intratumoral injection that included magnetic iron oxide (Fe3O4) nanoparticles and doxorubicin co-encapsulated in PLGA nanocarriers.
Oleic acid-coated Fe3O4 nanoparticles (4-6 nm) and doxorubicin were encapsulated in PLGA nanoparticles (200-300 nm) using the standard oil-in-water emulsion evaporation process. The nanoparticles were then delivered directly into the tumor core in a mouse Lewis lung cancer model [55]. An external magnetic field kept the nanoparticles in situ, allowing for regulated medication release and preventing loss to systemic circulation.
The magnetic field was produced with a 0.5 Tesla disk magnet. This was fastened to the skin right above the tumor and kept in place for 48 hours following injection. There was a significant decrease in tumour size in the group of animals treated with magnetic nanoparticles as compared to the control groups treated without a magnetic field and free medication.
Furthermore, no symptoms of systemic toxicity, such as weight loss, were found in the doxorubicin magnetic nanoparticle group established an inductive hyperthermia approach for solid lung tumors via intratumoral injection of magnetic nanoparticles [56]. A novel substance, ferucarbotran, was employed to achieve hyperthermia. It is made up of dextran and ultrafine (7 nm) magnetite nanoparticles. Theferucarbotran was given as a single dosage into the tumor core, followed by the use of an external alternating magnetic field to raise the intratumoral temperature to 43-45 degrees.
Direct medication instillation for locoregional therapy
The simplest method of loco-regional distribution is to inject chemotherapeutic solutions/suspensions directly into solid tumors (IT delivery) or the surrounding area. This is now used in a small variety of clinical indications, however there is little evidence of benefit [57].
Retinoblastoma
Retinoblastoma is a pediatric eye cancer, and intra-arterial chemotherapy for local delivery to the eye was initially proposed in the 1950s and 60s. Since the late 2000s, this approach has been refined to become the first and second line of therapy at several premier treatment facilities across the world.
Selective ocular artery infusion with melphalan, topotecan, or carboplatin solutions has been shown to enhance globe salvage rates and boost local concentrations by 10 - 30 fold when compared to IV chemotherapy [58]. However, the absence of solid randomized clinical trials to support the use of intraarterial chemotherapy has made it difficult to prove therapeutic superiority, especially when comparing metastasis rates after treatment.
It has been proposed that this sort of loco-regional therapy is more suited for individuals with advanced illness, and further research is needed to develop a solid evidence foundation for this treatment.
Peritoneal cancers
Hyperthermic intraperitoneal chemotherapy (HIPEC), also known as early postoperative intraperitoneal chemotherapy (EPIC), is a type of loco-regional medication administration that is now being utilized in clinical trials to treat advanced ovarian and other peritoneal malignancies. Cancers that extend to the peritoneal cavity have a very bad prognosis, with systemic chemotherapy having little impact due to insufficient blood flow to the peritoneal surface and hence little penetration into the tumor.
Surgical debulking can relieve symptoms but has minimal survival effects. Intraperitoneal chemotherapy allows for direct contact between the tumor surface and cytotoxic medicines, resulting in a local impact at the intended site of action [59].
Following surgical cytoreduction, a chemotherapeutic solution is pumped into the peritoneal cavity at an increased temperature of 42-43°C for 30-60 minutes.
The EPIC regimen is a five-day course of intraperitoneal chemotherapy following surgery. HIPEC now uses a variety of chemotherapeutic drugs, including alkylating agents (cisplatin, oxaliplatin, and carboplatin) and antitumor antibiotics (doxorubicin, mitomycin C).
The EPIC treatment included the antimetabolite 5-Flourouracil as well as taxane alkaloids. While several studies have shown that HIPEC treatment improves overall survival (OS) and progression-free survival (PFS) in total populations compared to groups that do not receive HIPEC treatment, clinical adoption has been limited due to toxicity concerns, resulting in increased morbidity and mortality [60].
Hepatocellular Carcinoma (HCC)
Since the 1980s, ethanol ablation in Hepatocellular Carcinoma (HCC) has been used to treat tiny tumors (<3cm) locally. It entails injecting 70% ethanol directly into the tumor while employing imaging guidance to follow the solution’s distribution throughout the tumor, and it was regarded as an efficacious, cost-effective method for these lesions due to its minimally invasive nature and low morbidity rate.
However, there were some limitations to its usage, including a limitation to early-stage HCC, inhomogeneous distribution inside the tumor, failure to reach tumor margins, and inadequate retention at the essential site of action. Because of the high likelihood of local recurrence, treatment regimens often include four to eight treatments once or twice each week [61].
Local recurrence rates of up to 33% have been reported for tumors less than 3cm, with rates increasing for bigger tumors. These concerns, along with developments in drug delivery technology, have confined the use of ethanol ablation to patient populations that are inappropriate for alternative therapies.
Bladder cancer
Bacillus Calmette-Guérin (BCG) has been used for over 40 years to treat superficial bladder cancer. It is administered intravenously, and the bladder’s hollow structure, with direct access via the urethra, makes it appropriate for this form of locoregional treatment. Antitumor drugs are localized to the desired site of action, reducing, but not eliminating, hazardous systemic effects and their usefulness in this application is well documented [62].
While the mechanism of action is not entirely known, it is connected to immune activation, which improves tumor cell detection and subsequent death via non-specific and specific cell-mediated pathways, and so represents an early example of immunotherapy.
Skin cancers
Because of the ease with which melanoma and nonmelanoma skin malignancies can be treated, IL injection has been extensively studied. Similar to bladder cancer, IL injection of BCG for melanoma was initially described in 1974, and, like bladder cancer and ethanol therapy in HCC, IL BCG needed many treatment cycles.
In the early years of usage, the data foundation for its use in melanoma was inadequate, and ECOG 1673, published in 2004, found no survival difference between treatment arms, demonstrating a lack of effectiveness associated with BCG use in melanoma [63]. This, along with adverse effects such as injection site response, seroconversion, and systemic infection, has led to a decline in the use of BCG in melanoma.
Other cancers
In addition to these normal clinical uses, there have been and continue to be a few additional tumors where the loco-regional distribution of chemotherapeutic solutions is utilized or has been studied therapeutically. Chemotherapeutics are administered intrathecal or intraventricularly to treat Leptomeningeal metastases (LM), a malignancy of the membranes that line the brain and spinal cord [64]. Intrathecal chemotherapy (ITC) using liposomal cytarabine (DepoCyt®), methotrexate, or thiotepa in conjunction with systemic treatment has been most widely employed; nevertheless, clinical effectiveness in comparison to systemic treatment alone is not conclusive.
Liposomal cytarabine has been unavailable in the clinic since 2017 owing to manufacturing concerns. In lung cancer, IT delivery of cisplatin to lung tumors and associated lymph nodes has been examined and demonstrated to be practical and safe, with a potential for enhanced survival, albeit this finding should be regarded with care due to the limited sample sizes. IT delivery has also been tested for palliative purposes in lung cancer [65].
A multi-center trial investigated the effects of IT administration of paratoluenesulfonamide in ethanol for palliation and debulking of severe malignant airway blockage and discovered that it increased survival with minimum normal tissue harm. There have also been a few trials in pancreatic cancer that investigated direct IT administration of gemcitabine using Endoscopic Ultrasound Fine Needle Injection (EUS-FNI), which established technical feasibility and safety but were not designed to assess effectiveness.
Loco-regional medication delivery in oncology: Current clinical assessment
Current clinical trials regarding loco-regional drug delivery can be classified into three categories: loco- regional delivery approaches using existing gold standard drugs alone or in combination with established delivery platforms, loco-regional delivery approaches using existing gold standard drugs in combination with new delivery platforms, along loco-regional delivery of newer classes of immune modulating therapies [66].
Chemotherapeutic drugs can be delivered locally or through established delivery channels
Most clinical studies employing current gold-standard chemotherapeutics involve changes to indications, treatment methods, or regimens. TACE evaluation in HCC is an active topic, with over 100 ongoing and recruiting studies exploring new combinations of beads/drugs or new therapy regimens. Beyond HCC, intra-arterial drug administration is on the rise, with 57 ongoing and recruiting studies in gliomas, neuroendocrine and pancreatic malignancies, and retinoblastoma [67]. Many of these trials concentrate on the use of novel intraarterial delivery devices, such as Surefire® (NCT02853500) and Renovocath® (NCT03257033), while others are exploring the use of bead technologies in therapeutic applications other than HCC.
A variety of presently licensed therapies, including temozolomide (NCT01180816), bevacizumab (NCT02285959), cetuximab (NCT02861898), and ipilimumab (NCT03233152), are being clinically studied for the treatment of HGGs by IA or IT. Trastuzumab (NCT01373710) and nivolumab (NCT03025256) are being studied for the treatment of LM, with case series and preliminary results indicating some potential.
At the time of writing, a review of clinicaltrials.gov revealed that there are currently 70 active and recruiting studies evaluating HIPEC treatment for a variety of peritoneal cancers, including ovarian, gastric, and colorectal, with a focus on different treatment regimens and/or different drug combinations [68].
Chemotherapeutic drugs can be delivered locally and through novel delivery systems
The development of drug delivery systems for loco-regional delivery in oncology applications has resulted in a plethora of formulations, platforms, and drug combinations that have been tested in pre-clinical trials across a wide range of tumor indications, including lung,
glioblastoma, ovarian, prostate, kidney, and pancreatic. However, despite this intensive research emphasis, only a small number of formulations have advanced to first place in human investigations [69].
Liproca®
Liproca® Depot (LIDSS Pharma) employs the Nanozolid® drug-delivery technology for the loco-regional therapy of prostate cancer, delivering 2-hydroxyflutamide for up to six months. Nanozolid® consists of compressed calcium sulfate granules combined with non-compressed calcium sulfate hemihydrate powder, which is rehydrated at the point of application to produce an injectable paste. The drug content in the non-compressed powder produces an early burst release, whereas the granule- encapsulated medication has a longer release time. Radiopaque calcium sulfate facilitates intra-procedural imaging of locoregional delivery. The combined findings of two Phase I/II single-arm interventional clinical studies (NCT00913263 & NCT02341404) (n=47) revealed that therapy was generally well tolerated after intra-prostatic injection of drug-loaded paste [70].
However, the clinical response varied throughout the patient group, indicating that this drug delivery platform’s therapeutic dosage and distribution require additional optimization to demonstrate meaningful clinical benefit. Based on the effectiveness results of these clinical studies, a third clinical study is being conducted to evaluate the potential use of Liproca® Depot during active monitoring of persons at high risk of prostate cancer progression (NCT03348527).
Other ways for local administration of anticancer medications
Beyond the aforementioned loco-regional techniques, little research has been conducted in the area of creating innovative strategies for administering nanopharmaceuticals for loco-regional therapy of lung cancer. Nonetheless, some other loco-regional platforms that have recently been investigated for the treatment of cancerous lesions that are not localized to the lung should be mentioned here, as they have the potential to be used in the future for nanopharmaceutical applications in lung cancers.
One especially successful loco-regional method has been the creation of wafers that can be implanted into the surgical cavity during high-grade gliomas. GliadelÕwafers are a commercially available delivery platform for carmustine, which is utilized as an adjuvant treatment for high-grade gliomas [71].
A 2011 systematic review outlines the clinical use of this therapeutic technique and evaluates its efficacy. Further research into comparable therapeutic techniques may play an important role in the loco-regional therapy of lung malignancies. Polymer films and electrospun composite materials are an exciting extension of traditional implant systems loaded with anticancer medicines.
These delivery systems may have an important role in future lung cancer therapy, particularly when combined with nanopharmaceuticals. A Chinese study group from Binzhou Medical University offered an example of this combination, incorporating docetaxel-loaded carbon nanotubes (DOX-CNT) into a composite nanofiber scaffold of PLGA by electrospinning [72].
The nanofibers generated were uniform and smooth, with widths ranging from 500 to 800 nm, and the integration of DOX-CNT contributed to the sustained release, with 50% doxorubicin released in 15 days, resulting in considerable anticancer effectiveness in vitro against HeLa cells. Most significantly, the excellent mechanical qualities of these nanofiber mats make them effective as a therapeutic patch for local anticancer drug delivery in surgical situations.
This delivery technique might have a considerable impact on the treatment of lung malignancies, particularly those with diffuse tumors and a poor prognosis following surgical resection, such as mesothelioma. Such a film might be placed intrapleurally after tumor excision or debulking to administer long-term, targeted chemotherapy to prevent local recurrence. The advantages of such a formulation arise mostly from its ability to further spatially localize and regulate drug release rates beyond the nanoparticle’s architecture [73].
Direct use of devices for sustained release of nanoencapsulated anticancer treatments offers enormous potential in the loco-regional therapy of lung cancer following surgical tumor removal. There is currently no data from in vivo studies of this application for lung cancer, however, considering the successes of clinical devices such as Gliadel wafers and favorable results from devices for the delivery of nanoparticles in vitro, it can be expected that this will be a keen area of research in the future [74]. Throughout the 28-day research, treated animals had a substantial reduction in tumor volume compared to their control group.
A key flaw in the study’s design was the use of an external magnetic field that had to be positioned 2 cm from the center of the tumor, which may be relevant in mice but not in any tumor that is not superficial in humans Table 1.
NCT no. | CT Phase | Title | Technology | Platform |
3145558 | 2 | TATE vs. TACE for Intermediate Stage HCC | TACE | Lipiodol and GelFoa. |
3268499 | 2 | TACE Emulsion vs. Suspension | TACE | Lipiodol |
2856126 | 3 | Comparing HAIC Plus Sorafenib (orally) vs TACE Plus Sorafenib (orally) for Advanced HCC | TACE HAIC | Lipiodol and PVA beads |
3116984 | 3 | A study comparing TACE with and without EBRT for advanced HCC (TACE-EBRT) | TACE EBRT | Lipiodol and gelatin sponge |
3192644 | 3 | Adjuvant TAI vs. TACE for Patients with HCC and PVTT after Hepatectomy: Effect on Recurrence Risk | TAI TACE | Lipiodol |
3727633 | 2 | HCC on Cirrhosis within Child A/B7 with HAI Injection of Idarubicin/Lipiodol Emulsion (LIDA-BII) | IA | lipiodol |
3107416 | 1/2 | To treat liver cancer, a diuretic is delivered into the artery and then blocked to starve the cells | HAI | n.s |
183885 | 2 | A Phase II study on IA Chemotherapy without Cisplatin and Mitomycin-C in Patients WA Phase II Study of IA Chemotherapy with Cisplatin and Mitomycin-C for Hepatocellular Carcinoma Patients | IA | n/a |
However, based on the findings of these two studies, intratumorally delivered magnetic nanotherapies appear to be worthy of further investigation in order to investigate their potential role in the future of lung cancer therapy, especially given that hyperthermia treatment has recently been made clinically viable [75].
Expert opinion
While there has been an increase in both scientific interest and clinical translation of loco-regional distribution in recent years, there have been few examples of its inclusion into routine treatment. Even TACE, the most frequently used locoregional therapy in HCC, lacks a strong consensus on its usage. This is partly due to a lack of emphasis on adequate delivery platform design and successful clinical trial design [78].
The sheer amount of elements to examine, including treatment groups, treatment regimens, medication selection and combinations, dose, and timing of treatments, has proven to be a considerable obstacle in achieving conclusive clinical outcomes. This has resulted to great variety in trial design, with huge numbers of trials, dominated by small patient numbers, posing a problem in reaching consensus on ideal procedures [79].
Clinical translation of loco-regional medication delivery necessitates the capacity to reach the tumor via minimally invasive procedures and image the delivery in real-time to guide therapy. Furthermore, retaining the drug formulation at the site of delivery is a significant difficulty, since fast drug clearance from the tumor site can result in erroneous and unpredictable dosage, as well as toxicity to surrounding tissue.
Finally, given the multi-modality approach to cancer treatment, the timing and placement of loco-regional treatment in the overall therapeutic program is complicated. Despite these obstacles, there is evidence that loco-regional therapeutic techniques are increasingly being used as part of overall treatment regimens in select solid tumor indications. Collaboration between clinicians and material scientists focussing on improved delivery platforms, as well as increased focus on clinical trial design, fuelled in particular by the immunotherapy space, is driving this resurgence in interest, increasing the number of products focused on clinical translation [80].
Furthermore, advances in medical device design and imaging modalities increase clinical accessibility, delivery, and monitoring, which is critical not just for clinical trials to assess delivery, retention, and efficacy, but also for clinical practice. Each tumor is distinct, and without suitable access and imaging guidance, loco-regional distribution is often not possible.
These advancements in a variety of scientific and medical specializations provide up prospects in a variety of indications, including those where loco-regional distribution is currently employed, such as HIPEC, TACE, and HGGs, as well as novel indications like pancreatic cancer. Evidence of this collaborative approach to treatment can be found in a variety of sources, including a recent review paper by Tabet et al [81].
That examined lessons learned compared to the clinical use of local and systemic adjuvant chemotherapy and discussed how these can inform the development of next-generation therapies. In addition, there appears to be an increased focus on assessing innovative therapeutic and delivery techniques for loco-regional distribution, including genes, miRNA, and siRNAs, with a special emphasis in pre-clinical investigations on nanoparticle formulations [82].
However, the use of loco-regional administration to improve and optimize immunotherapies, in particular, appears to have tremendous potential for extending therapeutic indications, boosting effectiveness, and lowering toxicity. Systemic immune treatment has demonstrated considerable immune-related adverse effects associated with a range of variables, and intratumoral administration can elicit an immune response locally, lowering toxicity and enhancing responsiveness in ‘cold’ tumors [83].
Ad d i t i o n a l l y, i m m u n o t h e r a p i e s p o se pharmacoeconomic problems. These medicines are exceedingly expensive, and if effective outcomes grow, so will the demand for their usage, posing a dilemma for healthcare systems. Local distribution can minimize dosage and related costs. Currently, the majority of IT immunotherapies in clinical trials are given by viral vectors or as simple injections of therapeutic in saline.
However, there is a growing corpus of pre-clinical research addressing a variety of IT or IL delivery techniques and platforms, including biodegradable micro and nanoparticles made from chitosan and alginate, emulsions, liposomes, lipids, and microneedles [84].
These systems have the potential to optimize the release profile and retention of molecules at the tumor site, allowing for more optimal treatment results. To summarise, loco-regional distribution is unlikely to provide stand-alone cancer therapy options, but it does have substantial potential as an adjuvant treatment in some of the most difficult-to-treat malignancies, presenting prospects for improved clinical outcomes in these patient populations [85].
In conclusion, Loco-regional medication distribution is a promising strategy for improving the efficacy and safety of cancer treatment. Despite existing obstacles like as drug resistance and tumor heterogeneity, new advances in nanoparticle formulations, hydrogels, and image-guided delivery approaches provide significant prospects to improve treatment results.
The future of cancer treatment depends on overcoming these challenges through novel techniques, such as integrating various therapeutic modalities and using nanotechnology for precise drug targeting. Collaboration among researchers, physicians, and industry partners is essential for implementing these advances in clinical practice.
By resolving present restrictions and capitalizing on new technology, loco-regional medication delivery has the potential to revolutionize cancer therapy, providing patients with more effective and personalized therapeutic alternatives.
Ethics Approval and Consent to Participate
Not applicable.
Consent for Publication
Not applicable.
Competing Interests
The authors declare no conflicts of interest.
Funding
This research received no specific grants from any funding agency in the public, commercial, or not-for- profit sector.
Acknowledgements
I would like to thank the SCHS-Sanskriti College of Higher Education and Studies, Kanpur Dehat, Uttar Pradesh, India, for motivating and helping us by providing the facilities required to write this review article.
Availability of Data and Materials
The data and supporting information are available within the article.
Author’s Contributions
PS makes a substantial contribution to conception and design. PS participated in the data analysis and interpretation. PS and MG acquired data. PS wrote the paper with input from all the authors. MG drawn the diagrams. All the authors have read and approved the final manuscript.
References
- Circulating DNA in patients undergoing loco-regional treatment of colorectal cancer metastases: a systematic review and meta-analysis Callesen LB , Takacova T, Hamfjord J, Würschmidt F, Oldhafer KJ , Brüning R, Arnold D, Spindler KG . Therapeutic Advances in Medical Oncology.2022;14. CrossRef
- Emerging strategies in developing multifunctional nanomaterials for cancer nanotheranostics Ang MJY , Chan SY , Goh Y, Luo Z, Lau JW , Liu X. Advanced Drug Delivery Reviews.2021;178. CrossRef
- Neoadjuvant Therapy for Breast Cancer as a Model for Translational Research Selli C, Sims AH . Breast Cancer: Basic and Clinical Research.2019;13. CrossRef
- Nano-drug delivery system for pancreatic cancer: A visualization and bibliometric analysis Zhao J, Zou F, Zhu J, Huang C, Bu F, Zhu Z, Yuan R. Frontiers in Pharmacology.2022;13. CrossRef
- Neoadjuvant Chemotherapy for Breast Cancer: Past, Present, and Future Asaoka M, Gandhi S, Ishikawa T, Takabe K. Breast Cancer: Basic and Clinical Research.2020;14. CrossRef
- Tumor microenvironment, immune response and post-radiotherapy tumor clearance Koukourakis M. I., Giatromanolaki A.. Clinical & Translational Oncology: Official Publication of the Federation of Spanish Oncology Societies and of the National Cancer Institute of Mexico.2020;22(12). CrossRef
- A phase II trial of the FGFR inhibitor pemigatinib in patients with metastatic esophageal-gastric junction/gastric cancer trastuzumab resistant: the FiGhTeR trial Merz V, Zecchetto C, Simionato F, Cavaliere A, Casalino S, Pavarana M, Giacopuzzi S, et al . Therapeutic Advances in Medical Oncology.2020;12. CrossRef
- Locoregional cancer therapy using polymer-based drug depots Ramazani F, Nostrum CF , Storm G, Kiessling F, Lammers T, Hennink WE , Kok RJ . Drug Discovery Today.2016;21(4). CrossRef
- Loco-regional treatment of hepatocellular carcinoma Lencioni R. Hepatology (Baltimore, Md.).2010;52(2). CrossRef
- Loco-regional cancer drug therapy: present approaches and rapidly reversible hydrophobization (RRH) of therapeutic agents as the future direction Budker VG , Monahan SD , Subbotin VM . Drug Discovery Today.2014;19(12). CrossRef
- Local drug delivery strategies for cancer treatment: gels, nanoparticles, polymeric films, rods, and wafers Wolinsky JB , Colson YL , Grinstaff MW . Journal of Controlled Release: Official Journal of the Controlled Release Society.2012;159(1). CrossRef
- Anticancer drug delivery with nanoparticles Conti M, Tazzari V, Baccini C, Pertici G, Serino LP , De Giorgi U. In Vivo (Athens, Greece).2006;20(6A).
- Loco-Regional and Systemic Chemotherapies for Hepato-Pancreatic Tumors: Integrated Treatments Ranieri G, Laface C. Cancers.2020;12(10). CrossRef
- Loco-regional interventional treatment of hepatocellular carcinoma: techniques, outcomes, and future prospects Lencioni R, Crocetti L, De Simone P, Filipponi F. Transplant International: Official Journal of the European Society for Organ Transplantation.2010;23(7). CrossRef
- Drug delivery systems for cancer drugs Martini A, Ciocca C. Expert Opinion on Therapeutic Patents.2005;13. CrossRef
- Potential advantages of loco-regional intra-arterial chemotherapy Cagol PP, Pasqual E, Bacchetti S. In vivo (Athens, Greece).2006;20(6A).
- Loco-regional radiosensitizing nanoparticles-in-gel augments head and neck cancer chemoradiotherapy Bhardwaj P, Gota V, Vishwakarma K, Pai V, Chaudhari P, Mohanty B, Thorat R, et al . Journal of Controlled Release: Official Journal of the Controlled Release Society.2022;343. CrossRef
- Preclinical evaluation of gene delivery methods for the treatment of loco-regional disease in breast cancer Rajendran S, O'Hanlon D, Morrissey D, O'Donovan T, O'Sullivan GC , Tangney M. Experimental Biology and Medicine (Maywood, N.J.).2011;236(4). CrossRef
- New Loco Regional Approaches to Treat Liver Cancer Afaq S, Soni JM , Pillai AK . Liver Diseases: A Multidisciplinary Textbook.2020;:687-94. CrossRef
- Clinical Considerations of Focal Drug Delivery in Cancer Treatment Harris J, Klonoski SC , Chiu B. Current Drug Delivery.2017;14(5). CrossRef
- Does local drug delivery still hold therapeutic promise for brain cancer? A systematic review Bastiancich C., Bozzato E., Henley I., Newland B.. Journal of Controlled Release: Official Journal of the Controlled Release Society.2021;337. CrossRef
- Nanotherapeutic systems for local treatment of brain tumors Chakroun RW , Zhang P, Lin R, Schiapparelli P, Quinones-Hinojosa A, Cui H. Wiley Interdisciplinary Reviews. Nanomedicine and Nanobiotechnology.2018;10(1). CrossRef
- Anticancer drug-loaded hydrogels as drug delivery systems for the local treatment of glioblastoma Bastiancich C., Danhier P., Préat V., Danhier F.. Journal of Controlled Release: Official Journal of the Controlled Release Society.2016;243. CrossRef
- Local strategies and delivery systems for the treatment of malignant gliomas Yu F, Asghar S, Zhang M, Zhang J, Ping Q, Xiao Y. Journal of Drug Targeting.2019;27(4). CrossRef
- Localized delivery of immunotherapeutics: A rising trend in the field Wang J, Zhang Y, Pi J, Xing D, Wang C. Journal of Controlled Release: Official Journal of the Controlled Release Society.2021;340. CrossRef
- Long-acting therapeutic delivery systems for the treatment of gliomas Padmakumar S, Amiji MM . Advanced Drug Delivery Reviews.2023;197. CrossRef
- Novel therapeutics for brain tumors: current practice and future prospects El Demerdash N, Kedda J, Ram N, Brem H, Tyler B. Expert opinion on drug delivery.2020;17(1). CrossRef
- Implantable and long-lasting drug delivery systems for cancer treatment. InLong-acting drug delivery systems Pacheco C, Baião A, Sousa F, Sarmento B. Woodhead Publishing.2022;:129-162. CrossRef
- Intratumoral cancer chemotherapy and immunotherapy: opportunities for nonsystemic preoperative drug delivery Goldberg Eugene P., Hadba Ahmad R., Almond Brett A., Marotta James S.. The Journal of Pharmacy and Pharmacology.2002;54(2). CrossRef
- Treatment of Malignant Brain Tumors with Controlled-Release Local-Delivery Polymers Kwon GS . InPolymeric Drug Delivery Systems.2005;:pp. 347-404. CRC Press..
- Biodegradable brain-penetrating DNA nanocomplexes and their use to treat malignant brain tumors Mastorakos P, Zhang C, Song E, Kim YE , Park HW , Berry S, Choi WK , et al . Journal of Controlled Release: Official Journal of the Controlled Release Society.2017;262. CrossRef
- Depot system for controlled release of gold nanoparticles with precise intratumoral placement by permanent brachytherapy seed implantation (PSI) techniques Lai P, Lechtman E, Mashouf S, Pignol J, Reilly RM . International Journal of Pharmaceutics.2016;515(1-2). CrossRef
- Biodegradable polymers for targeted delivery of anti-cancer drugs Doppalapudi S, Jain A, Domb AJ , Khan W. Expert Opinion on Drug Delivery.2016;13(6). CrossRef
- Intratumoral injection of hydrogel-embedded nanoparticles enhances retention in glioblastoma Brachi G, Ruiz-Ramírez J, Dogra P, Wang Z, Cristini V, Ciardelli G, Rostomily RC , et al . Nanoscale.2020;12(46). CrossRef
- Locoregional cancer treatment with magnetic drug targeting Alexiou C., Arnold W., Klein R. J., Parak F. G., Hulin P., Bergemann C., Erhardt W., Wagenpfeil S., Lübbe A. S.. Cancer Research.2000;60(23).
- Pancreatic Cancer: Challenges and Opportunities in Locoregional Therapies Bazeed AY , Day CM , Garg S. Cancers.2022;14(17). CrossRef
- OncoGel (ReGel/paclitaxel)--clinical applications for a novel paclitaxel delivery system Elstad NL , Fowers KD . Advanced Drug Delivery Reviews.2009;61(10). CrossRef
- Locoregional drug delivery using image-guided intra-arterial drug eluting bead therapy Lewis AL , Dreher MR . Journal of Controlled Release: Official Journal of the Controlled Release Society.2012;161(2). CrossRef
- Liposomal cancer chemotherapy: current clinical applications and future prospects Harrington K. J.. Expert Opinion on Investigational Drugs.2001;10(6). CrossRef
- Nanomedicine for Treatment of Lung Cancer Hussain S. Advances in Experimental Medicine and Biology.2016;890. CrossRef
- Nanomedicine-based adjuvant therapy: a promising solution for lung cancer Xu Y, Hsu JC , Xu L, Chen W, Cai W, Wang K. Journal of Nanobiotechnology.2023;21(1). CrossRef
- Advances in Lung Cancer Treatment Using Nanomedicines Sharma A, Shambhwani D, Pandey S, Singh J, Lalhlenmawia H, Kumarasamy M, Singh SK , et al . ACS omega.2023;8(1). CrossRef
- Pulmonary delivery of nanoparticle chemotherapy for the treatment of lung cancers: challenges and opportunities Mangal S, Gao W, Li T, Zhou QT . Acta Pharmacologica Sinica.2017;38(6). CrossRef
- Nanomedicine Combats Drug Resistance in Lung Cancer Zheng X, Song X, Zhu G, Pan D, Li H, Hu J, Xiao K, et al . Advanced Materials (Deerfield Beach, Fla.).2024;36(3). CrossRef
- Inhaled chemotherapy in lung cancer: future concept of nanomedicine Zarogoulidis P, Chatzaki E, Porpodis K, Domvri K, Hohenforst-Schmidt W, Goldberg EP , Karamanos N, Zarogoulidis K. International Journal of Nanomedicine.2012;7. CrossRef
- Innovative strategy for treatment of lung cancer: targeted nanotechnology-based inhalation co-delivery of anticancer drugs and siRNA Taratula O, Garbuzenko OB , Chen AM , Minko T. Journal of Drug Targeting.2011;19(10). CrossRef
- Inhalation treatment of lung cancer: the influence of composition, size and shape of nanocarriers on their lung accumulation and retention Garbuzenko OB , Mainelis G, Taratula O, Minko T. Cancer Biology & Medicine.2014;11(1). CrossRef
- Inhalable nanotherapeutics to improve treatment efficacy for common lung diseases Anderson CF , Grimmett ME , Domalewski CJ , Cui H. Wiley Interdisciplinary Reviews. Nanomedicine and Nanobiotechnology.2020;12(1). CrossRef
- siRNA Conjugated Nanoparticles-A Next Generation Strategy to Treat Lung Cancer Itani R, Al Faraj A. International Journal of Molecular Sciences.2019;20(23). CrossRef
- Current role of nanoparticles in the treatment of lung cancer Carrasco-Esteban E, Domínguez-Rullán JA , Barrionuevo-Castillo P, Pelari-Mici L, Leaman O, Sastre-Gallego S, López-Campos F. Journal of Clinical and Translational Research.2021;7(2).
- Fabrication of aerosol-based nanoparticles and their applications in biomedical fields Gautam M, Kim JO , Yong CS . Journal of Pharmaceutical Investigation.2021;51(4). CrossRef
- Inhaled chemotherapy adverse effects: mechanisms and protection methods Sardeli C, Zarogoulidis P, Kosmidis C, Amaniti A, Katsaounis A, Giannakidis D, Koulouris C, et al . Lung Cancer Management.2020;8(4). CrossRef
- Clinical experimentation with aerosol antibiotics: current and future methods of administration Zarogoulidis P, Kioumis I, Porpodis K, Spyratos D, Tsakiridis K, Huang H, Li Q, et al . Drug Design, Development and Therapy.2013;7. CrossRef
- (PDF) Aerosol-based Pulmonary Delivery of Therapeutic Molecules from Food Sources: Delivery Mechanism, Research Trends, and the Way Forward Lavanya MN , Preethi R, Moses JA , Anandharamakrishnan C. Food Reviews International.2022;38(sup1):753-88. CrossRef
- Aerosol-based fabrication of modified chitosans and their application for gene transfection Byeon JH , Kim H, Thompson DH , Roberts JT . ACS applied materials & interfaces.2014;6(7). CrossRef
- Nebulizers for drug delivery to the lungs Martin AR , Finlay WH . Expert Opinion on Drug Delivery.2015;12(6). CrossRef
- Jet, Ultrasonic, and Mesh Nebulizers: An Evaluation of Nebulizers for Better Clinical Outcomes Ari A. Euras J Pulm.2014;16. CrossRef
- Lipid nanocapsules: ready-to-use nanovectors for the aerosol delivery of paclitaxel Hureaux J, Lagarce F, Gagnadoux F, Vecellio L, Clavreul A, Roger E, Kempf M, et al . European Journal of Pharmaceutics and Biopharmaceutics: Official Journal of Arbeitsgemeinschaft Fur Pharmazeutische Verfahrenstechnik e.V.2009;73(2). CrossRef
- Aerosol Delivery of siRNA to the Lungs. Part 2: Nanocarrier-based Delivery Systems Youngren-Ortiz SR , Gandhi NS , España-Serrano L, Chougule MB . Kona: Powder Science and Technology in Japan.2017;34. CrossRef
- Poly(amidoamine) dendrimer nanocarriers and their aerosol formulations for siRNA delivery to the lung epithelium Conti DS , Brewer D, Grashik J, Avasarala S, Rocha SRP . Molecular Pharmaceutics.2014;11(6). CrossRef
- PAMAM dendrimers as aerosol drug nanocarriers for pulmonary delivery via nebulization Nasr M, Najlah M, D'Emanuele A, Elhissi A. International Journal of Pharmaceutics.2014;461(1-2). CrossRef
- Nanoparticle formulations in pulmonary drug delivery Bailey MM , Berkland CJ . Medicinal Research Reviews.2009;29(1). CrossRef
- Formulation and nebulization of fluticasone propionate-loaded lipid nanocarriers Umerska A, Mouzouvi CRA , Bigot A, Saulnier P. International Journal of Pharmaceutics.2015;493(1-2). CrossRef
- Aerosol delivery of nanoparticles in uniform mannitol carriers formulated by ultrasonic spray freeze drying D'Addio SM , Chan JGY , Kwok PCL , Benson BR , Prud'homme RK , Chan H. Pharmaceutical Research.2013;30(11). CrossRef
- Nanocarriers as pulmonary drug delivery systems to treat and to diagnose respiratory and non respiratory diseases Smola M, Vandamme T, Sokolowski A. International Journal of Nanomedicine.2008;3(1).
- Colloidal nanocarriers: a review on formulation technology, types and applications toward targeted drug delivery Mishra B., Patel BB , Tiwari S. Nanomedicine: Nanotechnology, Biology, and Medicine.2010;6(1). CrossRef
- Aerosol delivery of ferulic acid-loaded nanostructured lipid carriers: A promising treatment approach against the respiratory disorders Hassanzadeh P, Arbabi E., Rostami F, Atyabi F, Dinarvand R. Physiology and Pharmacology (Iran).2017;21.
- Formulation and clinical perspectives of inhalation-based nanocarrier delivery: a new archetype in lung cancer treatment Bardoliwala D, Javia A, Ghosh S, Misra A, Sawant K. Therapeutic Delivery.2021;12(5). CrossRef
- Locoregional therapies for hepatocellular carcinoma: a critical review from the surgeon's perspective Poon RT , Fan S, Tsang FH , Wong J. Annals of Surgery.2002;235(4). CrossRef
- Locoregional Therapy: Cancer Interventions with and Without Radionuclides Yevich S, Mahvash A. Locoregional Radionuclide Cancer Therapy: Clinical and Scientific Aspects.2021;:89-109. CrossRef
- Locoregional therapies and surgical oncology. InNew Technologies in Surgical Oncology 2010 (pp. 113-128). Milano: Springer Milan Rossi CR , Comandone A, Veltri A. . CrossRef
- Innovative therapies: intraoperative intracavitary chemotherapy Chang MY , Sugarbaker DJ . Thoracic Surgery Clinics.2004;14(4). CrossRef
- Multimodality locoregional treatment strategies for bridging HCC patients before liver transplantation Györi GP , Felsenreich DM , Silberhumer GR , Soliman T, Berlakovich GA . European surgery: ACA: Acta chirurgica Austriaca.2017;49(5). CrossRef
- Aerosolization of Nanotherapeutics as a Newly Emerging Treatment Regimen for Peritoneal Carcinomatosis Shariati M, Willaert W, Ceelen W, De Smedt SC , Remaut K. Cancers.2019;11(7). CrossRef
- Electromotive Enhanced Drug Administration in Oncology: Principles, Evidence, Current and Emerging Applications Min JWS , Saeed N, Coene A, Adriaens M, Ceelen W. Cancers.2022;14(20). CrossRef
- Prospective, randomized, double-blind, multi-center, Phase III clinical study on transarterial chemoembolization (TACE) combined with Sorafenib versus TACE plus placebo in patients with hepatocellular cancer before liver transplantation - HeiLivCa [ISRCTN24081794] Hoffmann K., Glimm H., Radeleff B., Richter G., Heining C., Schenkel I., Zahlten-Hinguranage A., et al . BMC cancer.2008;8. CrossRef
- Sorafenib or placebo plus TACE with doxorubicin-eluting beads for intermediate stage HCC: The SPACE trial Lencioni R, Llovet JM , Han G, Tak WY , Yang J, Guglielmi A, Paik SW , et al . Journal of Hepatology.2016;64(5). CrossRef
- Intraperitoneal chemotherapy for peritoneal metastases: an expert opinion Ceelen W, Braet H, Ramshorst G, Willaert W, Remaut K. Expert Opinion on Drug Delivery.2020;17(4). CrossRef
- Locoregional surgical and interventional therapies for advanced colorectal cancer liver metastases: expert consensus statements Abdalla EK , Bauer TW , Chun YS , D'Angelica M, Kooby DA , Jarnagin WR . HPB: the official journal of the International Hepato Pancreato Biliary Association.2013;15(2). CrossRef
- Drug delivery to the lymphatic system: importance in future cancer diagnosis and therapies Xie Y, Bagby TR , Cohen M. S., Forrest ML . Expert Opinion on Drug Delivery.2009;6(8). CrossRef
- DC Bead embolic drug-eluting bead: clinical application in the locoregional treatment of tumours Lewis AL , Holden RR . Expert Opinion on Drug Delivery.2011;8(2). CrossRef
- Recent Advances in Locoregional Therapy of Hepatocellular Carcinoma Podlasek A, Abdulla M, Broering D, Bzeizi K. Cancers.2023;15(13). CrossRef
- Locoregional treatments for hepatocellular carcinoma: Current evidence and future directions Inchingolo R, Posa A, Mariappan M, Spiliopoulos S. World Journal of Gastroenterology.2019;25(32). CrossRef
- Drug delivery embolization systems: a physician's perspective Nicolini A, Crespi S, Martinetti L. Expert Opinion on Drug Delivery.2011;8(8). CrossRef
- Locoregional therapies for metastatic colorectal carcinoma to the liver--an evidence-based review Xing M, Kooby DA , El-Rayes BF , Kokabi N, Camacho JC , Kim HS . Journal of Surgical Oncology.2014;110(2). CrossRef
License

This work is licensed under a Creative Commons Attribution-NonCommercial 4.0 International License.
Copyright
© Asian Pacific Journal of Cancer Biology , 2025
Author Details